
the
effects of altitude
the atmosphere
circulatory system
respiratory system
hypoxia
hyperventilation
pressure change effects on the body
Human beings are not
physiologically equipped for high altitudes. To cope, we must rely on
preventive measures and, in some cases, life-support equipment. Although
Army aviation primarily involves rotary-wing aircraft flying at
relatively low altitudes, aircrews may still encounter
altitude-associated problems. These may cause hypoxia, hyperventilation,
and trapped-gas and evolved-gas disorders. By understanding the
characteristics of the atmosphere, aircrews are better prepared for the
physiological changes that occur with increasing altitudes.
physical characteristics
of the atmosphere
The atmosphere is
like an ocean of air that surrounds the surface of the Earth. It is a
mixture of water and gases. The atmosphere extends from the surface of
the Earth to about 1,200 miles in space. Gravity holds the atmosphere
in place. The atmosphere exhibits few physical characteristics;
however, it shields the inhabitants of the Earth from ultraviolet
radiation and other hazards in space. Without the atmosphere, the
Earth would be as barren as the moon.
structure of the
atmosphere
The atmosphere
consists of several concentric layers, each displaying its own unique
characteristics. Each layer is known as a sphere. Thermal variances
within the atmosphere help define these spheres, offering aviation
personnel an insight into atmospheric conditions within each area.
Between each of the spheres is an imaginary boundary, known as a
pause.
the troposphere
The troposphere
extends from sea level to about 26,405 feet over the poles to nearly
52,810 feet above the equator. It is distinguished by a relatively
uniform decrease in temperature and the presence of water vapour,
along with extensive weather phenomena.
Temperature
changes in the troposphere can be accurately predicted using a
mean-temperature lapse rate of -1.98 degrees Celsius per 1,000 feet.
Temperatures continue to decrease until the rising air mass achieves
an altitude where temperature is in equilibrium with the surrounding
atmosphere. Table 2-1 illustrates the mean lapse rate and the pressure
decrease associated with ascending altitude.
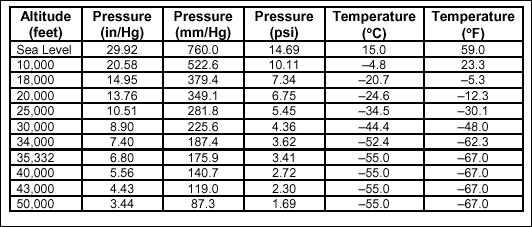
Table 2-1. Standard Pressure
and Temperature Values at 40 Degrees Latitude for Specific Altitudes
the stratosphere
The
stratosphere extends from the tropopause to about 158,430 feet (about
30 miles). The stratosphere can be subdivided based on thermal
characteristics found in different regions. Although these regions
differ thermally, the water-vapour content of both regions is
virtually nonexistent.
The first subdivision
of the stratosphere is termed the isothermal layer. In the isothermal
layer, temperature is constant at -55 degrees Celsius (-67 degrees
Fahrenheit). Turbulence, traditionally associated with the
stratosphere, is attributed to the presence of fast-moving jet
streams, both here and in the upper regions of the troposphere.
The second
subdivision of the stratosphere is characterized by rising
temperatures. This area is the ozonosphere. The ozonosphere serves as
a double-sided barrier that absorbs harmful solar ultraviolet
radiation while allowing solar heat to pass through unaffected. In
addition, the ozonosphere reflects heat from rising air masses back
toward the surface of the Earth, keeping the lower regions of the
atmosphere warm, even at night during the absence of significant solar
activity.
the mesosphere
The mesosphere
extends from the stratopause to an altitude of 264,050 feet (50
miles). Temperatures decline from a high of -3 degrees Celsius at the
stratopause to nearly -113 degrees Celsius at the mesopause.
Noctilucent
clouds are another characteristic of this atmospheric layer. Made of
meteor dust/water vapour and shining only at night, these cloud
formations are probably due to solar reflection.
the thermosphere
The
thermosphere extends from 264,050 feet (50 miles) to about 435 miles
above the Earth. The uppermost atmospheric region, the thermosphere is
generally characterized by increasing temperatures; however, the
temperature increase is in direct relation to solar activity.
Temperatures in the thermosphere can range from -113 degrees Celsius
at the mesopause to 1,500 degrees Celsius during periods of extreme
solar activity.
Another
characteristic of the thermosphere is the presence of charged ionic
particles. These particles are the result of high-speed subatomic
particles emanating from the sun. These particles collide with gas
atoms in the atmosphere and split them apart, resulting in a large
number of charged particles (ions).
composition of the atmosphere
The
atmosphere of the Earth is a mixture of gases. Although the atmosphere
contains many gases, few are essential to human survival. Those gases
required for human life are nitrogen, oxygen, and carbon dioxide.
Table 2-2 indicates
the percentage concentrations of gases commonly found in the
atmosphere.
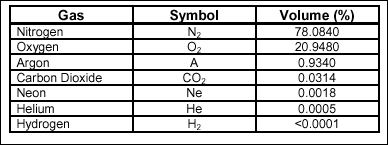
Table 2-2.
Percentages of Atmospheric Gases
nitrogen
The atmosphere of
the Earth consists mainly of nitrogen. Although a vital ingredient in
the chain of life, nitrogen is not readily used by the human body.
However, nitrogen saturates body fluids and tissues as a result of
respiration. Aircrews must be aware of possible evolved-gas disorders
because of the decreased solubility of nitrogen at higher altitudes.
oxygen
Oxygen is the second
most plentiful gas in the atmosphere. The process of respiration
unites oxygen and sugars to meet the energy requirements of the body.
The lack of oxygen in the body at altitude will cause drastic
physiological changes that can result in death. Therefore, oxygen is
of great importance to aircrew members.
carbon dioxide
Carbon
dioxide is the product of cellular respiration in most life forms.
Although not present in large amounts, the CO2
in the atmosphere plays a vital role in maintaining the oxygen supply
of the Earth. Through photosynthesis, plant life uses CO2
to create energy and releases O2 as a by-product. As a
result of animal metabolism and photosynthesis, CO2
and O2 supplies in the atmosphere remain constant.
other gases
Other gases—such as
argon, xenon, and helium—are present in trace amounts in the
atmosphere. They are not as critical to human survival as are
nitrogen, oxygen, and carbon dioxide.
atmospheric pressure
Standard
atmospheric pressure, or barometric pressure, is the force (that is,
weight) exerted by the atmosphere at any given point. An observable
characteristic, atmospheric pressure can be expressed in different
forms, depending on the method of measurement. Atmospheric pressure
decreases with increasing altitude, making barometric pressure of
great concern to aircrews because oxygen diffusion in the body depends
on total barometric pressure. Figure 2-1
illustrates the standard atmospheric pressure measurements at 59
degrees Fahrenheit (15 degrees Celsius) at sea level.
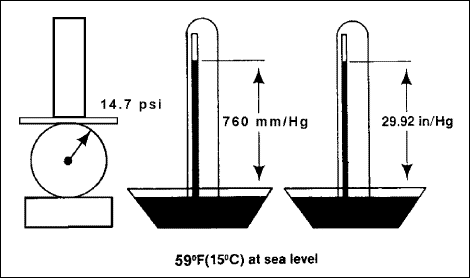
Figure
2-1. Standard Atmospheric Pressure Measurements at 59 Degrees Fahrenheit
(15 Degrees Celsius) at Sea Level
Dalton's law of partial pressures
A close
relationship exists between atmospheric pressure and the amount of the
various gases in the atmosphere. This relationship is referred to as
Dalton’s Law of Partial Pressures. Dalton’s Law states that the
pressure exerted by a mixture of ideal (non-reacting) gases is equal to
the sum of the pressures that each gas would exert if it alone
occupied the space filled by the mixture. The pressure of each gas
within a gaseous mixture is independent of the pressures of the other
gases in the mixture. The independent pressure of each gas is termed
the partial pressure of that gas. Figure 2-2 represents the concept of
Dalton’s Law as related to the atmosphere of the Earth.
Mathematically, Dalton’s Law can be expressed as follows:
Pt
= PN +
PO2 +
PCO2 + …
(constant volume and temperature)
Where
Pt represents the
total pressure of the mixture, PN,
PO2, PCO2,
… represent the partial pressures of each individual gas, V
represents volume, and T represents temperature. To determine
the partial pressure of the gases in the atmosphere (or any gaseous
mixture whose concentrations are known), the following mathematical
formula can be used:
Percentage of atmospheric |
concentration |
|
Total atmospheric |
of the
individual gas
100
|
x |
pressure at a given altitude = |
Partial pressure of the individual gas |
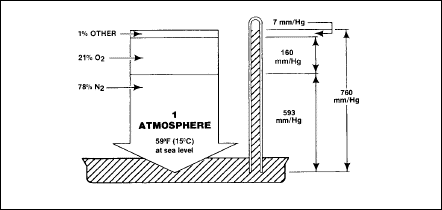
Figure
2-2. Dalton's Law of Partial Pressures as Related to the Atmosphere of the
Earth
Dalton’s Law
states that the pressure exerted by a mixture of ideal (nonreacting)
gases is equal to the sum of the pressures that each gas would exert
if it alone occupied the space filled by the mixture. The pressure of
each gas within a gaseous mixture is independent of the pressures of
the other gases in the mixture. The independent pressure of each gas
is termed the partial pressure of that gas. Figure 2-2 represents the
concept of Dalton’s Law as related to the atmosphere of the Earth.
For the
aircrew member, Dalton’s law illustrates that increasing altitude
results in a proportional decrease of partial pressures of gases found
in the atmosphere. Although the percentage concentration of gases
remains stable with increasing altitude, each partial pressure
decreases in direct proportion to the total barometric pressure. Table
2-3 shows the
relationship between barometric pressure and partial pressure.
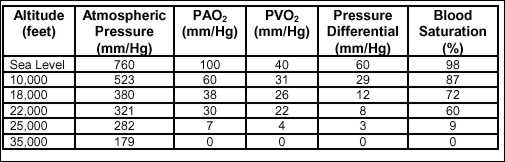
Table 2-3.
Partial Pressures of O2 at Various Altitudes
Changes in the
partial pressure of oxygen dramatically affect respiratory functions
within the human body. Any decrease in the partial pressure of oxygen
quickly results in physiological impairment. Although this impairment
may not be noticed initially at lower altitudes, the effects are
cumulative and grow progressively worse as altitude increases.
Decreases in
the partial pressure of nitrogen, especially at high altitude, can
lead to a decrease in the solubility of N2 in the body.
This decrease in N2 solubility can result in decompression
sickness.
physiological zones of the atmosphere
Humans are unable to
adapt physiologically to all of the physical changes that occur in the
different regions of the atmosphere. Because man evolved on the
surface, humans are especially susceptible to the dramatic temperature
and pressure changes that take place during ascent and sustained
aerial flight. Because of these factors, the atmosphere can be further
divided (by altitude) into three distinct physiological zones. These
divisions are primarily based on pressure changes that occur within
these parameters and the resultant effects on human physiology.
the efficient zone
Extending upward from sea level to 10,000 feet, the efficient zone
provides aircrews with a near-ideal physiological environment.
Although the barometric pressure drops from 760 mm/Hg at sea level to
523 mm/Hg at 10,000 feet, PO2
(partial pressure of oxygen) levels within this range allow humans to
operate in the efficient zone without using protective equipment;
however, sustained flight in the upper portions of this area may
require acclimatization. Some minor problems associated with the
efficient zone are ear and sinus blocks and gas expansion in the
digestive tract. Also, without the use of supplemental oxygen, a
decrease in night vision capabilities will occur above 4,000 feet.
the deficient zone
The deficient zone
of the atmosphere ranges from 10,000 feet at its base to 50,000 feet
at its highest point. Because atmospheric pressure at 10,000 feet is
only 523 mm/Hg, missions in the deficient zone carry a high degree of
risk unless supplemental-oxygen/cabin-pressurization systems are used.
As flights approach the upper limit of the deficient zone, decreasing
barometric pressures (down to 87 mm/Hg) make trapped-gas disorders
occur more frequently.
the space equivalent zone
Extending from
50,000 feet and continuing to the outer fringes of the atmosphere, the
space equivalent zone is totally hostile to human life. Therefore,
flight in the space equivalent zone requires a completely artificial
atmospheric environment. Unprotected exposure to the extremely low
temperatures and pressures found at these high altitudes can quickly
result in death. An example of how dangerous this area can be is found
at 63,000 feet (Armstrong’s line). The barometric pressure at this
altitude is only 47 mm/Hg, which equals the partial pressure of water
in the body. At this pressure, water begins to "boil" within the body
as it changes into a gaseous vapour.
structure and function of
the circulatory system
The circulatory
system, shown in Figure 2-3, constitutes the physiologic framework
required to transport blood throughout the body. A fundamental
function of the circulatory system (along with the lymphatic system)
is fluid transport. Other important functions of this system include
meeting body cell nutrition and excretion demands, along with
body-heat and electrochemical equilibrium requirements. Circulatory
components include arteries, capillaries, and veins that stretch to
nearly every cell in the body.
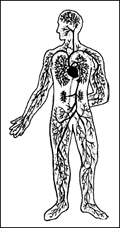
Figure
2-3. Structures of the Circulatory System
arteries
Conducting blood
away from the ventricles of the heart, the arteries are strong,
elastic vessels that can withstand relatively high pressures. Arterial
vessels generally carry oxygen-rich blood to the capillaries for use
by the tissues.
capillaries
The body’s
smallest blood vessels, the capillaries, form the junction between the
smallest arteries (arterioles) and the smallest veins (venules).
Actually semi permeable extensions of the inner linings of the
arterioles and venules, the capillaries provide body tissues with
access to the bloodstream. Capillaries can be found virtually
everywhere in the body, providing needed gas-/nutrient-exchange
capabilities to nearly every body cell.
veins
Transporting blood
from the capillaries back to the atria of the heart, the veins are the
blood-return portion of the circulatory system. A low-pressure
pathway, the veins also possess flap-like valves that ensure that
blood flows only in the direction of the heart. In addition, the veins
can constrict or dilate, based on the body’s requirements. This unique
ability allows blood flow and pressure to be modified, based on such
factors as body heat or trauma.
components and functions
of the blood
Although blood
volume varies with body size, the average adult has a blood volume
approaching 5 litres. About 5 percent of total body weight, blood is
actually a form of connective tissue whose cells are suspended in a
liquid intercellular material. The cellular portions of the blood
compose about 45 percent of blood volume and consist mainly of red
blood cells, white blood cells, and blood platelets. The remaining 55
percent of the blood is a liquid called plasma. Each of these
components performs unique functions, summarized in Figure 2-4.
red blood cells
Most of the
body’s supply of oxygen is transported by the red blood cells
(erythrocytes). Because oxygenation of red blood cells depends on the
Po2 in the atmosphere, aircrews may begin to suffer from
oxygen deficiency (hypoxia) even at low altitudes. RBC structure,
appearance, and production are among the factors that are affected
when erythrocytes experience hypoxia.
Haemoglobin
makes up about one-third of every red blood cell. Composed of several
polypeptide chains and iron-containing haeme groups, haemoglobin
attracts oxygen molecules through an electrochemical magnetic process.
Just as opposing poles on a magnet attract, so does the iron content
(Fe2+) within haemoglobin attract oxygen (O22-).
When the blood
supply is fully saturated with oxygen, as in arterial blood, blood
takes on a bright-red colour as oxyhaemoglobin is formed. As blood
passes through the capillaries, it releases oxygen to the surrounding
tissues. As a result, deoxyhaemoglobin forms and gives venous blood a
dark-red colour.
Red blood cells
are produced in the red bone marrow. The number of RBCs in circulating
blood is relatively stable; however, environmental factors play a
large role in determining the actual RBC count. Smoking, an inadequate
diet, and the altitude where one lives all contribute to fluctuations
in RBC count. In fact, people residing above 10,000 feet may have up
to 30 percent more erythrocytes than those living at sea level.
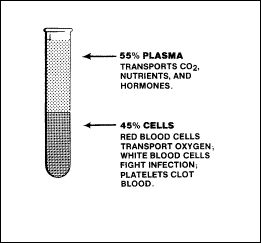
Figure
2-4. Functions of Blood Components
white blood cells
The principal
role of the white blood cells, or leukocytes, is to fight/control
various disease conditions, especially those caused by invading micro-organisms. Although WBCs are typically larger than RBCs, WBCs can
squeeze between the cells of blood vessels to reach diseased tissues.
WBCs also help form natural immunities against numerous disease
processes.
platelets
Although not
complete cells, the platelets, or thrombocytes, arise from small,
fragmented portions of much larger cells produced in the red bone
marrow. About half the size of an RBC, the platelets react to any
breach in the circulatory system through initialization of blood
coagulation and blood-vessel contraction.
plasma
The liquid portion
of the blood is a translucent, straw-coloured fluid, known as plasma.
All of the cellular structures in the bloodstream are suspended in
this liquid. Composed mainly of water, plasma also contains proteins
and inorganic salts. Some of the important functions of the plasma are
to transport nutrients, such as glucose, and waste products, such as
carbon dioxide.
the processes of
breathing and respiration
All known living
organisms exchange gases with their environment. This gas exchange is
known as respiration. The processes of respiration are breathing,
external respiration, and internal respiration.
breathing
Breathing can be
described as a spontaneous, rhythmic mechanical process. Contraction
and relaxation of the respiratory muscles cause gases to move in and
out of the lungs, thereby providing the body a gaseous media for
exchange purposes.
external
respiration
External respiration
takes place in the alveoli of the lungs. Air, which includes oxygen,
is moved to the alveoli by the mechanical process of breathing. Once
in the alveolar sacs, oxygen diffuses from the incoming air into the
bloodstream. At the same time, carbon dioxide diffuses from the venous
blood into the alveolar sacs.
internal respiration
Internal respiration
includes the use of blood oxygen and carbon dioxide production by
tissue cells, as well as gas exchange between cells and the
surrounding fluid medium. These mechanisms, known as the metabolic
process, produce the energy needed for life.
functions of respiration
Respiration has several functions. It brings O2 into the
body, removes CO2
from the body, and helps maintain the temperature and the acid-base
balance of the body.
oxygen intake
The primary
function of respiration is the intake of O2. Oxygen enters
the body through the respiratory system and is transported within the
body through the circulatory system. All body cells require oxygen to
metabolize food material.
carbon dioxide removal
Carbon
dioxide is one of the by-products of the metabolic process. CO2
dissolves in the blood plasma, which then transports it from the
tissues to the lungs so that it can be released.
body / heat balance
Body temperature is
usually maintained within a narrow range (from 97 to 100 degrees
Fahrenheit). Evaporation of bodily fluids (such as perspiration) is
one method of heat loss that helps maintain body-heat balance. The
warm, moist air released during exhalation also aids in this process.
body chemical balance
A
delicate balance exists between the amounts of oxygen and carbon
dioxide in the body. The uptake of O2 and CO2
takes place through extensive chemical changes in the haemoglobin and
plasma of the blood. Disrupting these chemical pathways changes the
chemical balance of the body.
Under normal conditions, the measure of relative acidity or alkalinity
(pH level) within the body is 7.35 to 7.45. During respiration, the
partial pressure of carbon dioxide elevates, the acidity level
increases, and the pH value lowers to less than 7.3. Conversely, too
little CO2
causes the blood to become more alkaline and the pH value to rise.
Figure 2-5 shows
how the amount of carbon dioxide in the body affects the pH level of
the blood.
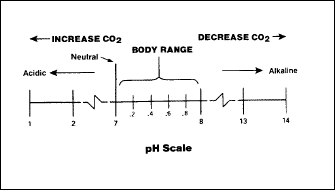
Figure 2-5. Relationship of CO2
Content and pH Level of the Blood
Because
the human body maintains equilibrium within narrow limits, the
respiratory centers of the brain sense any shift in the blood pH and
partial pressure of CO2
(PCO2) levels.
When unusual levels occur, chemical receptors trigger the respiratory
process to help return the PCO2
and pH levels to normal limits. The 7.2 to 7.6 limits are critical for
the necessary uptake of O2 by the blood and the release of
that O2 to tissues.
phases of external
respiration
The respiratory
cycle is an involuntary process that continues unless a conscious
effort is made to control it. External respiration occurs in two
phases: active (inhalation) and passive (exhalation). Figure 2-6
illustrates these phases.
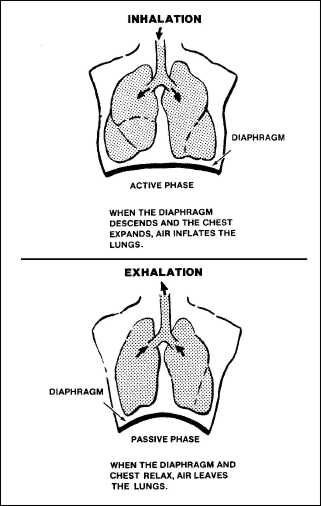
Figure
2-6. The Phases of Respiration
active phase (inhalation)
The movement of air
into the lungs is the active phase of external respiration, or
inhalation. It is caused by the expansion of the chest wall and
downward motion of the diaphragm. Inhalation creates an area of low
pressure because of the increased volume in the lungs. Because of the
greater outside pressure, air will then rush into the lungs to inflate
them.
passive phase
(exhalation)
In the
passive phase of external respiration, or exhalation, the diaphragm
relaxes and the chest wall contracts downward to create increased
pressure inside the lungs. Once the glottis opens, this pressure
inside the lungs causes the air to rush out, which frees CO2
to the atmosphere.
components of the
respiratory system
The respiratory
system consists of passages and organs that bring atmospheric air into
the body. The components of the respiratory system, shown in Figure
2-7, include the oral-nasal passage, pharynx, larynx, trachea,
bronchi, bronchioles, alveolar ducts, and alveoli.
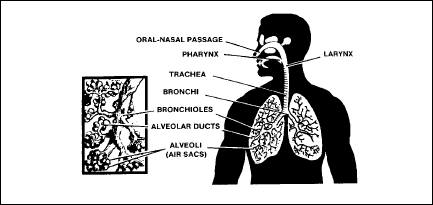
Figure
2-7. Components of the Respiratory System
oral / nasal passage
The oral-nasal
passage includes the mouth and nasal cavities. The nasal passages are
lined with a mucous membrane that contains many fine, ciliated hair
cells. The membrane’s primary purpose is to filter air as it enters
the nasal cavity. The hairs continually clean the membrane by sweeping
filtered material to the back of the throat where it is either
swallowed or expelled through the mouth. Therefore, air that enters
through the nasal cavity is better filtered than air that enters
through the mouth.
pharynx
The pharynx, the
back of the throat, is connected to the nasal and oral cavities. It
primarily humidifies and warms the air entering the respiratory
system.
trachea
The trachea, or
windpipe, is a tube through which air moves down into the bronchi.
From there, air continues to move down increasingly smaller passages,
or ducts, until it reaches the small alveoli within the lung tissue.
aveoli
Each
tiny alveolus is surrounded by a network of capillaries that joins
veins and arteries. The microscopic capillaries, each having a wall
only one cell in thickness, are so narrow that red blood cells move
through them in single file. The actual gaseous exchange between CO2
and O2 occurs in the alveoli.
Carbon dioxide and oxygen move in and out of alveoli because of the
pressure differentials between their CO2
and O2 levels and those in surrounding capillaries. This
movement is based on the law of gaseous diffusion: a gas always moves
from an area of high pressure to an area of lower pressure. Figure 2-8
illustrates the exchange of CO2
and O2 between an alveolus and a capillary.
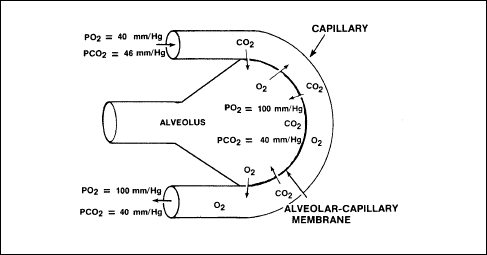
Figure 2-8. Diffusion of CO2
and O2 Between an Alveolus
and a Capillary
When O2
reaches the alveoli of the lungs, it crosses a thin cellular barrier
and moves into the capillary bed to reach the oxygen-carrying RBCs. As
the oxygen enters the alveoli, it has a partial pressure of oxygen of
about 100 mm/Hg. Within the blood, the Po2 of the venous
return blood is about 40 mm/Hg. As the blood traverses the capillary
networks of the alveoli, the O2 flows from the area of high
pressure within the alveoli to the area of low pressure within the
blood. Thus, O2 saturation takes place.
Carbon
dioxide diffuses from the blood to the alveoli in the same manner. The
partial pressure of carbon dioxide (PCO2)
in the venous return blood of the capillaries is about 46 mm/Hg, as
compared to a PCO2
of 40 mm/Hg in the alveoli. As the blood moves through the
capillaries, the CO2
moves from the high PCO2
in the capillaries to an area of lower PCO2
in the alveoli. The CO2
is then exhaled during the next passive phase (exhalation) of
respiration.
Note:
The exchange of O2 and CO2
between tissue and capillaries occurs in the same manner as it does
between the alveoli and capillaries.
The amount of O2 and CO2
transferred across the alveolar-capillary membrane into the blood
depends primarily on the alveolar pressure of oxygen in relation to
the venous pressure of oxygen. This pressure differential is critical
to the crew member because O2 saturation in the blood
decreases as altitude increases. This decrease in O2
saturation can lead to hypoxia, which is caused by a deficiency of O2
in the body tissues. Table 2-4 shows the relationship between altitude
and O2 saturation.
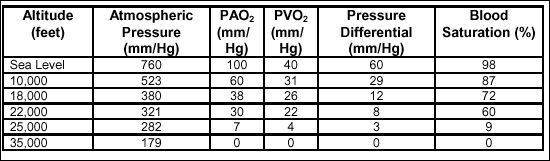
Table
2-4. Correlation of Altitude and Blood O2
Saturation
characteristics of hypoxia
Hypoxia results when
the body lacks oxygen. Hypoxia tends to be associated only with
flights at high altitude. However, many other factors—such as alcohol
abuse, heavy smoking, and various medications—interfere with the
blood’s ability to carry oxygen. These factors can either diminish the
ability of the blood to absorb oxygen or reduce the body’s tolerance
to hypoxia.
types
of hypoxia
There are four
major types of hypoxia: hypoxic, hypaemic, stagnant, and histotoxic.
They are classified according to the cause of the hypoxia.
hypoxic
hypoxia
Hypoxic hypoxia occurs when not enough oxygen is in the air or when
decreasing atmospheric pressures prevent the diffusion of O2
from the lungs to the bloodstream. Aviation personnel are most likely
to encounter this type at altitude. It is due to the reduction of the
PO2
at high altitudes, as shown in Figure 2-10.
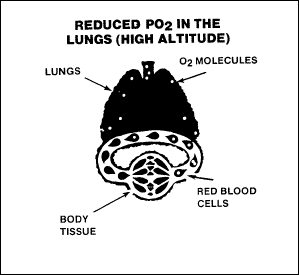
Figure
2-10. Hypoxic Hypoxia
hypaemic
hypoxia
Hypaemic, or
anaemic, hypoxia is caused by a reduction in the oxygen-carrying
capacity of the blood, as shown in Figure 2-11. Anaemia and blood loss
are the most common causes of this type. Carbon monoxide, nitrites,
and sulpha drugs also cause this hypoxia by forming compounds with
haemoglobin and reducing the haemoglobin that is available to combine
with oxygen.
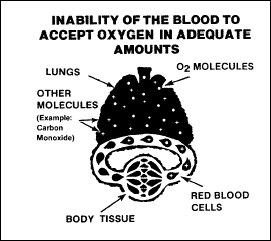
Figure
2-11. Hypaemic Hypoxia
stagnant
hypoxia
In stagnant hypoxia,
the oxygen-carrying capacity of the blood is adequate but, as shown in
Figure 2-12, circulation is inadequate. Such conditions as heart
failure, arterial spasm, and occlusion of a blood vessel predispose
the individual to stagnant hypoxia. More often, when a crew member
experiences extreme gravitational forces, disrupting blood flow and
causing the blood to stagnate.
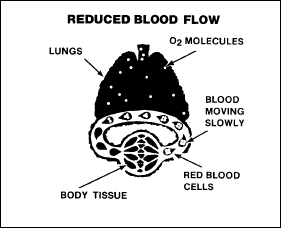
Figure
2-12. Stagnant Hypoxia
histotoxic
hypoxia
This type
results when there is interference with the use of O2 by
body tissues. Alcohol, narcotics, and certain poisons—such as
cyanide—interfere with the cells’ ability to use an adequate supply of
oxygen. Figure 2-13 shows the result of this oxygen deprivation.
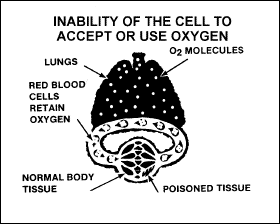
Figure
2-13. Histotoxic Hypoxia
signs, symptoms and
susceptibility to hypoxia
signs and symptoms
hypoxia
Signs are observable
by the other aircrew members and, therefore, are objective. Individual
aircrew members observe or feel their own symptoms. These symptoms
vary from one person to another and, therefore, are subjective.
Aviation personnel
commonly experience mild hypoxia at altitudes at or above 10,000 feet.
Those who fly must be able to recognize the possible signs and
symptoms. Being able to recognize these signs and symptoms is
particularly important because the onset of hypoxia is subtle and
produces a false sense of well-being. Crew members are often engrossed
in flight activities and do not readily notice the symptoms of
hypoxia. Usually, however, most individuals experience two or three
unmistakable symptoms or signs that cannot be overlooked. Figure 2-14
lists the signs and symptoms.
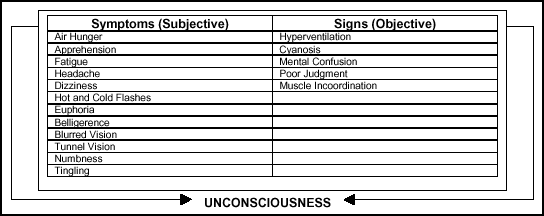
Figure
2-14. Possible Signs and Symptoms of Hypoxia
susceptibility to
hypoxia
Individuals vary
widely in their susceptibility to hypoxia. Several factors determine
individual susceptibility.
Onset Time and Severity
The onset time and
severity of hypoxia vary with the amount of oxygen deficiency. Crew
members must be able to recognize hypoxia and immediately determine
the cause.
Self-Imposed Stress
Physiological
Altitude. An individual’s physiological altitude, the altitude that
the body feels, is as important as the true altitude of a flight.
Self-imposed stressors, such as tobacco and alcohol, increase the
physiological altitude.
Smoking. The
haemoglobin molecules of RBCs have a 200- to 300-times greater affinity
for carbon monoxide than for oxygen. Cigarette smoking significantly
increases the amount of CO carried by the haemoglobin of RBCs; thus, it
reduces the capacity of the blood to combine with oxygen. Smoking 3
cigarettes in rapid succession or 20 to 30 cigarettes within 24 hours
before a flight may saturate from 8 to 10 percent of the haemoglobin in
the blood. The physiological effects of this condition include—
-
The loss of about 20
percent of the smoker’s night vision at sea level.
-
A physiological
altitude of 5,000 feet at sea level, as depicted in Figure 2-15.
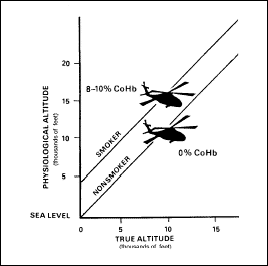
Figure
2-15. Adverse Effects of Altitude on Smokers
Individual Factors
Metabolic rate,
diet, nutrition, and emotions greatly influence an individual’s
susceptibility to hypoxia. These and other individual factors must be
considered in determining susceptibility.
Ascent Rate
Rapid ascent rates
affect the individual’s susceptibility to hypoxia. High altitudes can
be reached before the crew member notices serious symptoms.
Exposure Duration
The effects of
exposure to altitude relate directly to an individual’s length of
exposure. Usually, the longer the exposure, the more detrimental the
effects. However, the higher the altitude, the shorter the exposure
time required before symptoms of hypoxia occur.
Ambient Temperature
Extremes in
temperature usually increase the metabolic rate of the body. A
temperature change increases the individual’s oxygen requirements
while decreasing the tolerance of the body to hypoxia. With these
conditions, hypoxia may develop at lower altitudes than usual.
Physical Activity
When physical
activity increases, the body demands a greater amount of oxygen. This
increased oxygen demand causes a more rapid onset of hypoxia.
Physical Fitness
An individual who is
physically conditioned will normally have a higher tolerance to
altitude problems than one who is not. Physical fitness raises an
individual’s tolerance ceiling.
effects of hypoxia
In aviation, the
most important effects of hypoxia are those related, either directly
or indirectly, to the nervous system. Nerve tissue has a heavy
requirement for oxygen. Brain tissue is one of the first areas
affected by an oxygen deficiency. A prolonged or severe lack of oxygen
destroys brain cells. Hypoxia demonstrations in an altitude chamber do
not produce any known brain damage because the severity and duration
of the hypoxia are minimized.
The expected
performance time is from the interruption of the oxygen supply until
the crew member loses the ability to take corrective action. Table 2-5
shows that the EPT varies with the altitude at which the individual is
flying. An aircrew flying in a pressurized aircraft that loses cabin
pressurization, as in rapid decompression, has only one-half of the
EPT shown in Table 2-5.
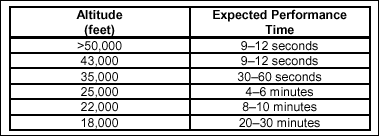
Table
2-5. Relationship Between Expected Performance Time and Altitude
stages of hypoxic hypoxia
There are four
stages of hypoxic hypoxia: indifferent, compensatory, disturbance, and
critical. Table 2-6 shows that the stages vary according to the
altitude and the severity of symptoms.
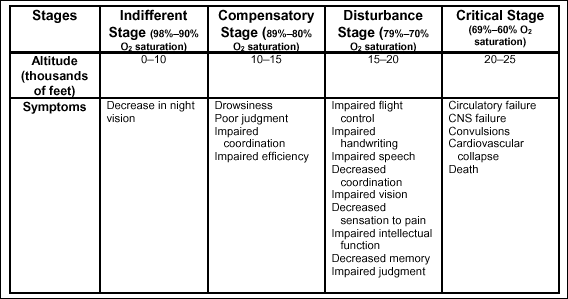
Table
2-6. Stages of Hypoxia
indifferent stage
Mild hypoxia in this
stage causes night vision to deteriorate at about 4,000 feet. Aircrew
members who fly above 4,000 feet at night should be aware that visual
acuity decreases significantly in this stage because of both the dark
conditions and the developing mild hypoxia.
compensatory stage
The
circulatory system and, to a lesser degree, the respiratory system
provide some defence against hypoxia at this stage. The pulse rate,
systolic blood pressure, circulation rate, and cardiac output
increase. Respiration increases in depth and sometimes in rate. At
12,000 to 15,000 feet, however, the effects of hypoxia on the nervous
system become increasingly apparent. After 10 to 15 minutes, impaired
efficiency is obvious. Crew members may become drowsy and make
frequent errors in judgment. They may also find it difficult to do
even simple tasks requiring alertness or moderate muscular
coordination. Crew members preoccupied with duties can easily overlook
hypoxia at this stage.
disturbance stage
In this stage, the
physiological responses can no longer compensate for the oxygen
deficiency. Occasionally, crew members become unconscious from hypoxia
without undergoing the subjective symptoms described in Table 2-6.
Fatigue, sleepiness, dizziness, headache, breathlessness, and euphoria
are the symptoms most often reported. The objective symptoms explained
below are also experienced.
Senses
Peripheral vision
and central vision are impaired, and visual acuity is diminished.
Weakness and loss of muscular coordination are experienced. The
sensations of touch and pain are diminished or lost. Hearing is one of
the last senses to be lost.
Mental Processes
Intellectual
impairment is an early sign that often prevents the individual from
recognizing disabilities. Thinking is slow, and calculations are
unreliable. Short-term memory is poor, and judgment—as well as
reaction time—is affected.
Personality Traits
There may be a
display of basic personality traits and emotions much the same as with
alcoholic intoxication. Euphoria, aggressiveness, overconfidence, or
depression can occur.
Psychomotor Functions
Muscular
coordination is decreased, and delicate or fine muscular movements may
be impossible. Stammering and illegible handwriting are typical of
hypoxic impairment.
Cyanosis
When cyanosis
occurs, the skin becomes bluish in colour. This effect is caused by
oxygen molecules failing to attach to haemoglobin molecules.
critical stage
Within three to five
minutes, judgment and coordination usually deteriorate. Subsequently,
mental confusion, dizziness, incapacitation, and unconsciousness
occur.
prevention of hypoxic
hypoxia
An
understanding of the causes and types of hypoxia assists in its
prevention. Hypoxic (altitude) hypoxia is the type most often
encountered in aviation. The other three types (hypaemic, stagnant,
and histotoxic) may also present danger to aviators.
Hypoxic hypoxia can
be prevented by ensuring that sufficient oxygen is available to
maintain an alveolar partial pressure of oxygen (PAO2) between 60 and
100 mm/Hg. Preventive measures include—
During night flights
above 4,000 feet, crew members should use supplemental oxygen when
available. Supplemental oxygen is necessary because of the mild
hypoxia and loss of visual acuity that occur.
The amount, or
percentage, of oxygen required to maintain normal oxygen saturation
levels varies with altitude. At sea level, a 21 percent concentration
of ambient air oxygen is necessary to maintain the normal blood oxygen
saturation of 96 to 98 percent. At 20,000 feet, however, a 49 percent
concentration of oxygen is required to maintain the same saturation.
The upper limit of
continuous-flow oxygen is reached at about 34,000 feet. Above 34,000
feet, positive pressure is necessary to maintain an adequate oxygen
saturation level. The positive pressure, however, cannot exceed 30
mm/Hg because—
-
Normal oxygen
masks cannot hold positive pressures of more than 25 mm/Hg without
leaking.
-
Excess pressure
may enter the middle ear through the Eustachian tubes and cause the
eardrum to bulge outward, which is painful.
-
Crew members
encounter difficulty in exhalation against the pressure, resulting
in hyperventilation.
Pressurization, as
found in the C-12 aircraft, can prevent hypoxia. Supplemental oxygen
should be available in the aircraft in case of pressurization loss.
The prevention
of hypoxic hypoxia is essential in the aviation environment. There
are, however, other causes of hypoxia. Carbon monoxide uptake (hypaemic
hypoxia), the effects of alcohol (histotoxic hypoxia), and reduced
blood flow (stagnant hypoxia) are also hazardous. Avoiding or
minimizing self-imposed stressors helps eliminate hypoxic conditions.
treatment of hypoxia
Individuals who
exhibit signs and symptoms of hypoxia must be treated immediately.
Treatment consists of giving the individual 100 percent oxygen. If
oxygen is not available, descent to an altitude below 10,000 feet is
mandatory. When symptoms persist, the type and cause of the hypoxia
must be determined and treatment administered accordingly.
characteristics of
hyperventilation
Hyperventilation is
the excessive rate and depth of respiration leading to abnormal loss
of carbon dioxide from the blood. This condition occurs more often
among aviators than is generally recognized. It seldom incapacitates
completely, but it causes disturbing symptoms that can alarm the
uninformed aviator. In such cases, an increased breathing rate and
anxiety then further aggravate the problem.
causes of
hyperventilation
The human body
reacts automatically under conditions of stress and anxiety whether
the problem is real or imaginary. Often, a marked increase in
breathing rate occurs. This increase leads to a significant decrease
in the carbon-dioxide content of the body as well as a change in the
acid-base balance. Among the factors that can initiate this cycle are
emotions, pressure breathing, and hypoxia.
emotions
When
fear, anxiety, or stress alters the normal breathing pattern, the
individual may attempt to consciously control breathing. The
respiration rate is then likely to increase without an elevation in CO2
production, and hyperventilation occurs.
pressure breathing
Positive-pressure
breathing is used to prevent hypoxia at altitude. It reverses the
normal respiratory cycle of inhalation and exhalation.
Inhalation
Under
positive-pressure conditions, the aviator is not actively involved in
inhalation as in the normal respiratory cycle. The aviator does not
inhale oxygen into the lungs; instead, oxygen is forced into the lungs
under positive pressure.
Exhalation
Under
positive-pressure conditions, the aviator is forced to breathe out
against the pressure. The force that the individual must exert in
exhaling results in an increased rate and depth of breathing. At this
point, too much CO2
is lost and alkalosis, or increased pH, occurs. Pauses between
exhaling and inhaling can reverse this condition and maintain a
near-normal level of CO2
during pressure breathing.
hypoxia
With the
onset of hypoxia and the resultant lower oxygen-saturation level of
the blood, the respiratory centre triggers an increase in the
breathing rate to gain more oxygen. This rapid breathing, which is
beneficial for oxygen uptake, causes excessive loss of carbon dioxide
when continued too long.
signs and symptoms of
hyperventilation
The
excessive loss of CO2
and the chemical imbalance that occur during hyperventilation produce
signs and symptoms. These include—
-
Dizziness.
-
Muscle spasms.
-
Unconsciousness.
-
Visual impairment.
-
Tingling
sensations.
-
Hot and cold
sensations.
The signs and
symptoms of hyperventilation and hypoxia are similar, making them
difficult to differentiate. The indications given below help to
distinguish between the two.
Hyperventilation
Hyperventilation
results in nerve and muscle irritability and muscle spasms. Symptoms
appear gradually.
Fainting
Fainting produces
loose muscles but no muscle spasms. Symptoms appear rapidly.
treatment of hyperventilation
The most effective
method of treatment is voluntary reduction in the affected
individual’s rate of respiration. However, an extremely apprehensive
person may not respond to directions to breathe more slowly.
Although
it is difficult, an individual affected by the symptoms of
hyperventilation should try to control the respiration rate; the
normal rate is 12 to 16 breaths per minute. To treat hyperventilation,
the aviator should control breathing and go to 100 percent oxygen. If
symptoms continue and conscious control of respiration is not
possible, the individual should talk or sing. It is physiologically
impossible to talk and hyperventilate at the same time. Talking or
singing will elevate the CO2
level and help regulate breathing.
When hypoxia
and hyperventilation occur concurrently, a decrease in the respiratory
rate and the intake of 100 percent O2 will correct the
condition. If hypoxia is severe, the aviator must return to ground
level before becoming incapacitated.
dysbasis
The human
body can withstand enormous changes in barometric pressure as long as
air pressure in the body cavities equals ambient air pressure.
Difficulty occurs when the expanding gas cannot escape so that ambient
and body pressures can equalize. The discussion in this section
applies to non-pressurized flight and direct exposure of aircrews to
potentially harmful altitudes.
Dysbarism
refers to the various manifestations of gas expansion induced by
decreased barometric pressure. These manifestations can be just as
dangerous, if not more so, than hypoxia or hyperventilation. The
direct effects of decreased barometric pressure can be divided into
two groups: trapped-gas disorders and evolved-gas disorders.
trapped gas disorders
During ascent, the
free gas normally present in various body cavities expands. If the
escape of the expanded volume is impeded, pressure builds up within
the cavity and pain is experienced. The expansion of trapped gases
accounts for abdominal pain, ear pain, sinus pain, or toothache.
Boyle's law
Trapped-gas problems
are explained by the physical laws governing the behaviour of gases
under conditions of changing pressure. Boyle’s Law (Figure 2-16)
states that the volume of a gas in inversely proportional to the
pressure exerted upon it. Differences in gas expansion are found under
conditions of dry gas and wet gas.
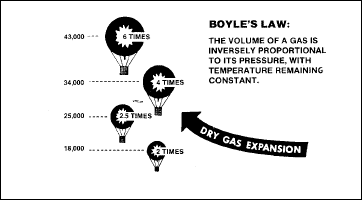
Figure
2-16. Boyle's Law
Dry-Gas Conditions
Under dry-gas
conditions, the atmosphere is not saturated with moisture. Under
conditions of constant temperature and increased altitude, the volume
of a gas expands as the pressure decreases.
Wet-Gas Conditions
Gases within
the body are saturated with water vapour. Under constant temperature
and at the same altitude and barometric pressure, the volume of wet
gas is greater than the volume of dry gas.
trapped gas disorders in
the gastrointestinal tract
With a rapid
decrease in atmospheric pressure, aircrews frequently experience
discomfort from gas expansion within the digestive tract. At low or
intermediate altitudes, the symptom is not serious in most
individuals. Above 25,000 feet, however, enough distension may occur
to produce severe pain. Figure 2-17 shows the dramatic expansion of
trapped gas as altitude increases.
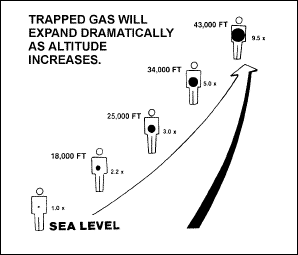
Figure
2-17. Trapped-Gas Expansion in the Gastrointestinal Tract at Increased
Altitudes
Cause
The stomach
and the small and large intestines normally contain a variable amount
of gas at a pressure roughly equal to the surrounding atmospheric
pressure. The stomach and large intestine contain considerably more
gas than does the small intestine. The chief sources of this gas are
swallowed air and, to a lesser degree, gas formed as a result of
digestive processes, fermentation, bacterial decomposition, and
decomposition of food undergoing digestion. The gases normally present
in the gastrointestinal tract are oxygen, carbon dioxide, nitrogen,
hydrogen, methane, and hydrogen sulphide. The proportions vary, but the
highest percentage of the gas mixture is always nitrogen.
Effects
The absolute volume
or location of the gas may cause gastrointestinal pain at high
altitude. Sensitivity or irritability of the intestine, however, is a
more important cause of gastrointestinal pain. Therefore, an
individual’s response to high altitude varies, depending on such
factors as fatigue, apprehension, emotion, and general physical
condition. Gas pains of even moderate severity may produce marked
lowering of blood pressure and loss of consciousness if distension is
not relieved. For this reason, any individual experiencing gas pains
at altitude should be watched for pallor or other signs of fainting.
If these signs are noted, an immediate descent should be made.
Prevention
Aircrews should
maintain good eating habits to prevent gas pains at high altitudes.
Some foods that commonly produce gas are onions, cabbages, raw apples,
radishes, dried beans, cucumbers, and melons. Crew members who
participate regularly in high-altitude flights should avoid foods that
disagree with them. Chewing the food well is also important. When
people drink liquids or chew gum, they unavoidably swallow air.
Therefore, crew members should avoid drinking large quantities of
liquids, particularly carbonated beverages, before high-altitude
missions and chewing gum during ascent. Eating irregularly, hastily,
or while working makes individuals more susceptible to gas pains. Crew
members who fly frequent, long, and difficult high-altitude missions
should be given special consideration in diet and in the environment
in which they eat. They should watch their diet, chew food well, and
keep regular bowel habits.
Relief
If trapped-gas
problems exist in the gastrointestinal tract at high altitude,
belching or passing flatus will ordinarily relieve the gas pains. If
pain persists, descent to a lower altitude is necessary.
trapped gas disorders of the ears
The ear is
not only an organ of hearing but also one of regulating equilibrium.
When ascending to altitude, aircrew members often experience
physiological discomfort during changes in atmospheric pressure. As
barometric pressure decreases during ascent, the expanding air in the
middle ear (Figure 2-18) is intermittently released through the
Eustachian tube (slender tube between the middle ear and the pharynx)
into the nasal passages. As the inside pressure increases, the eardrum
bulges until an excess pressure of about 12 to 15 mm/Hg is reached. At
this time, the air trapped in the middle ear is forced out of the
middle ear and the eardrum resumes its normal position. Just before
the air escapes into the Eustachian tube, there is a sensation of
fullness in the ear. As the pressure is released, there is often a
click or pop.
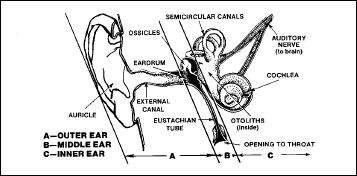
Figure
2-18. Anatomy of the Ear
Cause
During
flight. During descent, the change in pressure within the ear may
not occur automatically. Equalizing the pressure in the middle ear
with that of the outside air may be difficult. The Eustachian tube
allows air to pass outward easily but resists passage in the opposite
direction. With the increase in barometric pressure during descent,
the pressure of the external air is higher than the pressure in the
middle ear and the eardrum is pushed in (Figure 2-19). If the pressure
differential increases appreciably, it may be impossible to open the
Eustachian tube. This painful condition could cause the eardrum to
rupture because the Eustachian tube cannot equalize the pressure. When
the ears cannot be cleared, marked pain ensues. If the pain increases
with further descent, ascending to a level at which the pressure can
be equalized provides the only relief. Then a slow descent is
recommended. Descending rapidly from a level of 30,000 to 20,000 feet
will often cause no discomfort; a rapid descent from 15,000 to 5,000
feet, however, will cause great distress. The change in barometric
pressure is much greater in the latter situation. For this reason,
special care is necessary during rapid descents at low altitudes.
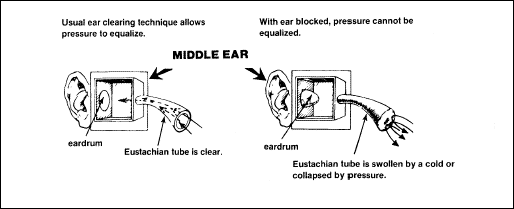
Figure
2-19. Pressure Effect on the Middle Ear During Descent
After
Flight. Crew members who have breathed pure oxygen during an
entire flight sometimes develop delayed ear block several hours after
landing, although their ears were cleared adequately during descent.
Delayed ear blocks are caused by saturation of the middle ear with
oxygen. After crew members return to breathing ambient air, the tissue
gradually reabsorbs the oxygen present in the middle ear. When a
sufficient amount is absorbed, the pressure in the ear becomes less
than that on the outside of the eardrum. Ear pain may awaken crew
members after they have gone to sleep, or they may notice it when they
awake the following morning. Usually this condition is mild and can be
relieved by performing the Valsalva manoeuvre explained in paragraph
2-130 below.
Complications From
Pre-existing Physical Conditions
Respiratory Infections. Crew members often complain of discomfort
in the ears caused by inability to ventilate the middle ear
adequately. Such inability occurs most frequently when the Eustachian
tube or its opening is swollen shut as the result of inflammation or
infection coincidental with a head cold, sore throat, infection of the
middle ear, sinusitis, or tonsillitis. In such cases, forceful opening
of the tube may cause a disease-carrying infection to enter the middle
ear along with the air. Therefore, crew members who have colds and
sore throats should not fly. If flight is essential, slow descents
will equalize pressure more easily.
Temporal
Bone and Jaw Problems. Although upper respiratory infections are
the main causes of narrowing of the Eustachian tube, there are other
causes. Crew members with malposition of the temporomandibular joint
(temporal bone and jaw) may have ear pain and difficulty both in
ventilating the middle ear and in hearing. In these cases, movement of
the jaw (or yawning) relaxes surrounding soft tissues and clears the
opening of the Eustachian tube.
Prevention and Treatment
During
Flight. Normally, crew members can equalize pressure during
descent by swallowing or yawning or by tensing the muscles of the
throat. If these methods do not work, they can perform the Valsalva
manoeuvre. To do this, they close the mouth, pinch the nose shut, and
blow sharply. This manoeuvre forces air through the previously closed
Eustachian tube in the cavity of the middle ear; pressure will
equalize. With repeated practice in rapidly clearing the ears, crew
members can more easily tolerate increased rates of descent.
Note: To avoid
over pressurization of the middle ear, crew members should never
attempt a Valsalva manoeuvre during ascent.
After
Flight. If middle-ear and ambient pressures have not equalized
after landing and the condition persists, aviation personnel should
consult a flight surgeon because barotitis media can occur. This is an
acute or chronic traumatic inflammation of the middle ear caused by a
difference of pressure on opposite sides of the eardrum. It is
characterized by congestion, inflammation, discomfort, and pain in the
middle ear and may be followed by temporarily or permanently impaired
hearing, usually the former.
trapped gas disorders of the sinuses
Like the
middle ear, sinuses can also trap gas during flight. The sinuses
(Figure 2-20) are air-filled, relatively rigid, bony cavities lined
with mucous membranes. They connect with the nose by means of one or
more small openings. The two frontal sinuses are within the bones of
the forehead; the two maxillary sinuses are within the cheekbones; and
the two ethmoid sinuses are within the bones of the nose.
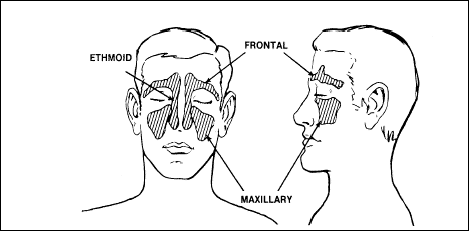
Figure
2-20. Sinus Cavities
Cause
If the openings into
the sinuses are normal, air passes into and out of these cavities
without difficulty and pressure equalizes during ascent or descent.
Swelling of the mucous membrane lining, caused by an infection or
allergic condition, may obstruct the sinus openings. Viscous
secretions that coat tissue may also cover the openings. These
conditions may make it impossible to equalize the pressure. Change of
altitude produces a pressure differential between the inside and the
outside of the cavity, sometimes causing severe pain. Unlike the ears,
ascent and descent almost equally affect the sinuses. If the frontal
sinuses are involved, the pain extends over the forehead above the
bridge of the nose. If the maxillary sinuses are affected, the pain is
on either side of the nose in the region of the cheekbones. Maxillary
sinusitis may produce pain in the teeth of the upper jaw; the pain may
be mistaken for toothache.
Prevention
As with
middle-ear problems, sinus problems are usually preventable. Aircrew
members should avoid flying when they have a cold or congestion.
During descent, they can perform the Valsalva maneuver often. The
opening to a sinus cavity is quite small, compared to the Eustachian
tube; unless the pressure is equalized, extreme pain will result. If
crew members notice any pain in a sinus on ascent, they should avoid
any further increase in altitude.
Treatment
If a sinus
block occurs during descent, aircrews should avoid further descent.
They should attempt a forceful Valsalva manoeuvre. If this manoeuvre
does not clear the sinuses, they should ascend to a higher altitude.
This ascent will ventilate the sinuses. They can also perform the
normal Valsalva manoeuvre during slow descent to the ground. If the
aircraft is equipped with pressure-breathing equipment, they can use
oxygen, under positive pressure, to ventilate the sinuses. If the
pressure does not equalize after landing, crew members should consult
the flight surgeon.
trapped gas disorders of
the teeth
Changes in
barometric pressure cause toothache, or barodontalgia. This is a
significant but correctable indisposition. The toothache usually
results from an existing dental problem. The onset of toothache
generally occurs from 5,000 to 15,000 feet. In a given individual, the
altitude at which the pain occurs shows a remarkable constancy. The
pain may or may not become more severe as altitude increases. Descent
almost invariably brings relief; the toothache often disappears at the
same altitude at which it first occurred.
evolved gas disorders
Evolved-gas
disorders occur in flight when atmospheric pressure is reduced as a
result of an increase in altitude. Gases dissolved in body fluids at
sea-level pressure are released from solution and enter the gaseous
state as bubbles when ambient pressure is lowered (Henry’s Law). This
will cause varied skin and muscle symptoms, which are sometimes
followed by neurological symptoms. Evolved-gas disorders are also
known as decompression sickness.
Henry's law
The amount of gas
dissolved in a solution is directly proportional to the pressure of
the gas over the solution. Henry’s Law is similar to the example of
gases being held under pressure in a soda bottle (Figure 2-21). When
the cap is removed, the liquid inside is subject to a pressure less
than that required to hold the gases in solution; therefore, gases
escape in the form of bubbles. Nitrogen in the blood is affected by
pressure changed in this same manner.
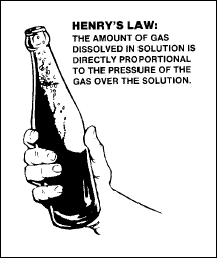
Figure
2-21. Henry's Law
Inert gases
in body tissues (principally nitrogen) are in equilibrium with the
partial pressures of the same gases in the atmosphere. When barometric
pressure decreases, the partial pressures of atmospheric gases
decrease proportionally. This decrease in pressure leaves the tissues
temporarily supersaturated. Responding to the super saturation, the
body attempts to establish a new equilibrium by transporting the
excess gas volume in the venous blood to the lungs.
cause
The cause of the
various symptoms of decompression sickness is not fully understood.
This sickness can be attributed to the nitrogen saturation of the
body. This is related, in turn, to the inefficient removal and
transport of the expanded nitrogen gas volume from the tissues to the
lungs. Diffusion to the outside atmosphere would normally take place
here.
Tissues and
fluid of the body contain from 1 to 1.5 litres of dissolved nitrogen,
depending on the pressure of nitrogen in the surrounding air. As
altitude increases, the partial pressure of atmospheric nitrogen
decreases and nitrogen leaves the body to re-establish equilibrium. If
the change is rapid, recovery of equilibrium lags, leaving the body
supersaturated. The excess nitrogen diffuses into the capillaries in
solution and is carried by the venous blood for elimination. With
rapid ascent to altitudes of 30,000 feet or more, nitrogen tends to
form bubbles in the tissues and in the blood. In addition to nitrogen,
the bubbles contain small quantities of carbon dioxide, oxygen, and
water vapour. Additionally, fat dissolves five or six times more
nitrogen than blood. Thus, tissues having the highest fat content are
more likely to form bubbles.
influential factors
Evolved-gas
disorders do not happen to everyone who flies. The following factors
tend to increase the chance of evolved-gas problems.
Rate of Ascent, Level of
Altitude, and Duration of Exposure
In general, the more
rapid the ascent, the greater the chance that evolved-gas disorders
will occur; the body does not have time to adapt to the pressure
changes. At altitudes below 25,000 feet, symptoms are less likely to
occur; above 25,000 feet, they are more likely to occur. The longer
the exposure, especially above 20,000 feet, the more likely that
evolved-gas disorders will occur.
Age and Body Fat
An increase in the
incidence of decompression sickness occurs with increasing age, with a
three-fold increase in incidence between the 19- to 25-year old and
the 40- to 45-year old age groups. The reason for this increase is not
understood but may result form the changes in circulation caused by
aging. No scientific validation exists to support any link between
obesity and the incidence of decompression sickness.
Physical Activity
Physical exertion
during flight significantly lowers the altitude at which evolved-gas
disorders occur. Exercise also shortens the amount of time that
normally passes before symptoms occur.
Frequency of Exposure
Types of
Evolved-Gas Disorders. Frequency of exposure tends to increase the
risk of evolved-gas disorders. The more often that individuals are
exposed to altitudes above 18,000 feet (without pressurization), the
more that they are predisposed to evolved-gas disorders.
Bends.
At the onset of bends, pain in the joints and related tissues may be
mild. The pain, however, can become deep, gnawing, penetrating, and
eventually, intolerable. The pain tends to be progressive and becomes
worse if ascent is continued. Severe pain can cause loss of muscular
power of the extremity involved and, if allowed to continue, may
result in bodily collapse. The pain sensation may diffuse from the
joint over the entire area of the arm or leg. In some instances, it
arises initially in muscle or bone rather than in a joint. The larger
joints, such as the knee or shoulder, are most frequently affected.
The hands, wrists, and ankles are also commonly involved. In
successive exposures, pain tends to recur in the same location. It may
also occur in several joints at the same time and worsens with
movement and weight bearing. Coarse tremors of the fingers are often
noted when the bends occur in joints of the arm.
Chokes.
Symptoms occurring in the thorax are probably caused, in part, by
innumerable small bubbles that block the smaller pulmonary vessels. At
first, a burning sensation is noted under the sternum. As the
condition progresses, the pain becomes stabbing and inhalation is
markedly deeper. The sensation in the chest is similar to one that an
individual experiences after completing a 100-yard dash. Short breaths
are necessary to avoid distress. There is an almost uncontrollable
desire to cough, but the cough is ineffective and non-productive.
Finally, there is a sensation of suffocation; breathing becomes more
shallow, and the skin turns bluish. When symptoms of chokes occur,
immediate descent is imperative. If allowed to progress, the condition
leads to collapse and unconsciousness. Fatigue, weakness, and soreness
in the chest may persist for several hours after the aircraft lands.
Paresthesia.
Tingling, itching, cold, and warm sensations are believed to be caused
by bubbles formed either locally or in the CNS where they involve
nerve tracts leading to the affected areas in the skin. Cold and warm
sensations of the eyes and eyelids, as well as occasional itching and
gritty sensations, are sometimes noted. A mottled red rash may appear
on the skin. More rarely, a welt may appear, accompanied by a burning
sensation. Bubbles may develop just under the skin, causing localized
swelling. Where there is excess fat beneath the skin in the affected
region, soreness accompanied by an abnormal accumulation of fluid may
be present for one or two days.
Central
Nervous System Disorders. In rare cases when aircrews are exposed
to high altitude, symptoms may indicate that the brain or the spinal
cord is affected by nitrogen-bubble formation. The most common
symptoms are visual disturbances such as the perception of lights as
flashing or flickering when they are actually steady. Other symptoms
may be a dull-to-severe headache, partial paralysis, the inability to
hear or speak, and the loss of orientation. Paresthesia or one-sided
numbness and tingling may also occur. Hypoxia and hyperventilation may
cause similar numbness and tingling; however, these are bilateral—they
occur in both arms, legs, or sides. CNS disorders are considered a
medical emergency; if they occur at high altitude, immediate descent
and hospitalization are indicated.
prevention
In high-altitude
flight and during hypobaric-chamber operations, aircrews can be
protected against decompression sickness. Protective measures include—
De-nitrogenation
Aircrews should breathe 100 percent oxygen for 30 minutes before takeoff
for flights above 18,000 feet. Denitrogenation rids the body of excess
nitrogen. This dumping of nitrogen from the body takes place because
no nitrogen is coming in via the oxygen mask under 100 percent oxygen.
The amount of nitrogen lost depends strictly on time. Within the first
30 minutes of denitrogenation (Figure 2-22), the body loses about 30
percent of its nitrogen.
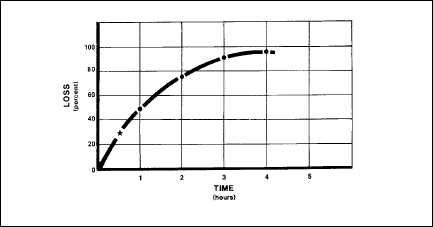
Figure
2-22. Nitrogen Elimination
Cabin Pressurization
The pressurized
aircraft cabin is usually maintained at a pressure equivalent to an
altitude of 10,000 feet or below. This pressure lessens the
possibility of nitrogen-bubble formation.
Limitation of Time at
High Altitude
The longer
one stays at high altitude, the more nitrogen bubbles will form.
Extended, un-pressurized flight above 20,000 feet should be minimized.
Aircrew Restrictions
Crew members should
not
for 24 hours after scuba diving. During scuba diving, excessive
nitrogen uptake by the body occurs while using compressed air. Flying
at 8,000 feet within 24 hours after scuba diving at 30 feet subjects
an individual to the same factors that a non-diver faces when flying
un-pressurized at 40,000 feet: nitrogen bubbles form.
treatment
When symptoms and
signs of evolved-gas disorders appear, aircrews should take the
following corrective actions:
-
Descend to ground
level immediately.
-
Place the affected
individual on 100 percent oxygen to eliminate any additional
nitrogen uptake and to remove excessive nitrogen from the system.
-
Immobilize the
affected area to prevent further movement of nitrogen bubbles in the
circulatory system.
-
Report to the
flight surgeon or to the best medical assistance available.
-
Undergo
compression therapy in a hyperbaric chamber if symptoms persist and
when prescribed by a flight surgeon.
delayed onset of
decompression sickness
The onset of
decompression sickness can occur as long as 48 hours after exposure to
altitudes above 18,000 feet. This delayed onset may occur even if no
signs/symptoms were evident during the flight.
|