
principles and problems of visionAircrew members rely more on the visual sense than any other sense to
orient themselves in flight. The following visual factors contribute to
aviation performance: good depth perception for safe landings, good
visual acuity to identify terrain features and obstacles in the flight
path, and good colour vision. Although vision is the most accurate and
reliable sense, visual cues can be misleading, contributing to incidents
occurring within the flight environment. Aviation personnel must be
aware of and know how to compensate effectively for the following:
physical deficiency or self-imposed stress, such as smoking, which
limits night-vision capability; visual-cue deficiencies; visual
limitations, consisting of degraded visual acuity, dark adaptation, and
colour and depth perception. For example, at night, the unaided eye has
degraded visual acuity. Aircrew members
must learn and effectively apply proper night-vision viewing techniques
to compensate for this limitation.
visual deficiencies
One contributing factor associated in achieving safe and
successful flights is that aviation personnel must be able to
recognize and understand common visual deficiencies. Important eye
problems related to degraded visual acuity and depth perception
include myopia, hyperopia, astigmatism, presbyopia, and retinal
rivalry. Surgical procedures to sculpt or reshape the cornea may also
result in visual deficiencies.
myopia
This condition, often referred to as nearsightedness, is
caused by an error in refraction in which the lens of the eye does not
focus an image directly on the retina. When a myopic person views an
image at a distance, the actual focal point of the eye is in front of
the retinal plane (wall), causing blurred vision. Thus, distant
objects are not seen clearly; only nearby objects are in focus. Figure 8-1 depicts this condition.
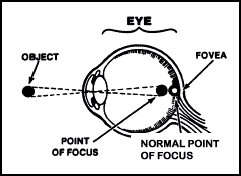
Figure 8-1. Myopia (Nearsightedness)
night
myopiaAt night, blue wavelengths of light prevail in the visible
portion of the spectrum. Therefore, slightly nearsighted (myopic)
individuals viewing blue-green light at night may experience blurred
vision. Even aircrew members with perfect vision will find that image
sharpness decreases as pupil diameter increases. For individuals with
mild refractive errors, these factors combine to make vision
unacceptably blurred unless they wear corrective glasses.
Another factor to consider is "dark focus." When light levels
decrease, the focusing mechanism of the eye may move toward a resting
position and make the eye more myopic. These factors become important
when aircrew members rely on terrain features during unaided night
flights. Special corrective lenses can be prescribed to correct for
night myopia.
hyperopia
Hyperopia is also caused by an error in refraction—the lens
of the eye does not focus an image directly on the retina. In a
hyperopic state, when an aircrew member views a near image, the actual
focal point of the eye is behind the retinal plane (wall), causing
blurred vision. Objects that are nearby are not seen clearly; only
more distant objects are in focus. This problem, referred to as
farsightedness, is shown in Figure 8-2.
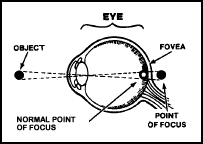
Figure 8-2. Hyperopia (Farsightedness)
astigmatism
An unequal curvature of the cornea or lens of the eye causes
this condition. A ray of light is spread over a diffuse area in one
meridian. In normal vision, a ray of light is sharply focused on the
retina. Astigmatism is the inability to focus different meridians
simultaneously. If, for example, astigmatic individuals focus on power
poles (vertical), the wires (horizontal) will be out of focus for most
of them, as shown in Figure 8-3.
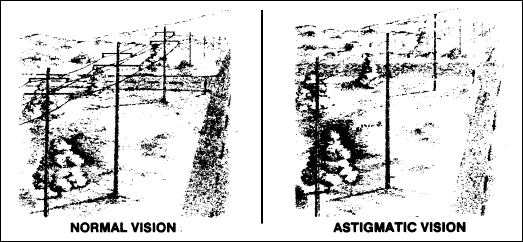
Figure 8-3. Astigmatism
presbyopia
This condition is part of the normal aging process, which
causes the lens to harden. Beginning in their early teens, the human
eye gradually loses the ability to accommodate for and focus on nearby
objects. When people are about 40 years old, their eyes are unable to
focus at normal reading distances without reading glasses. Reduced
illumination interferes with focus depth and accommodation ability.
Hardening of the lens may also result in clouding of the lens
(cataract formation). Aviators with early cataracts may see a standard
eye chart clearly under normal daylight but have difficulty seeing
under bright light conditions. This problem is due to the light
scattering as it enters the eye. This glare sensitivity is disabling
under certain circumstances. Glare disability, related to contrast
sensitivity, is the ability to detect objects against varying shades
of backgrounds. Other visual functions decline with age and affect the
aircrew member’s performance:
retinal rivalry
Eyes may experience this problem when attempting to
simultaneously perceive two dissimilar objects independently. This
phenomenon may occur when pilots view objects through the heads-up
display in the AH-64 Apache. If one eye views one image while the
other eye views another, conflict arises in total perception. Quite
often, the dominant eye will override the non-dominant eye, possibly
causing the information delivered to the non-dominant eye to be missed.
Additionally, this rivalry may lead to ciliary spasms and eye pain.
Mental conditioning and practice appear to alleviate this condition;
therefore, retinal rivalry becomes less of a problem as aircrew
members gain experience.
surgical procedures
Radial Keratotomy
Radial keratotomy is a surgical procedure that creates
multiple radial, lased, spokelike incisions through the use of an
argon laser upon the cornea of the eye to improve visual acuity.
Radial keratotomy permanently disqualifies an individual from flight
for Army aviation. The resulting glare sensitivity (sparkling effect
throughout the viewing field) and tissue scarring contribute to flight
disqualification.
Photorefractive Keratectomy
PRK is a procedure to correct corneal refractive errors by
use of a laser. The laser has replaced the scalpel in surgical
correction of myopia. PRK ablates or reshapes the central cornea. The
effects of this procedure flatten the cornea, which bends or refracts
the light properly on the retina, correcting the myopic deficiency.
This procedure is currently being considered for approval but, at this
time, like radial keratotomy, permanently disqualifies an individual
from flight duty for Army aviation. Irregularity of the cornea surface
causes astigmatism, the most common cause of disqualification.
LASIK or Keratomileusis
LASIK is the procedure used to carve and reshape the cornea.
Surgeons use a laser to shave the anterior half of the cornea,
creating a flap. The flap is retracted, and the inner side of the
cornea is reshaped with a laser, causing the cornea to flatten. When
the reshaping is completed, the flap is replaced in its original
position and sutured (sewn) back into place, similar to a Band-Aid®
effect. The flatter cornea now bends or refracts the light properly on
the retina. Unlike radial keratotomy or PRK, this technique can
correct for severe myopia and hyperopia. The main adverse effect is
irregularity of the corneal surface, causing astigmatism. In addition,
if the flap of an individual who has undergone this procedure became
suddenly unattached in an accident, the result would be a permanent
defect to the cornea and severely degraded visual acuity. There may be
serious effects which could disqualify the pilot from flying.
Various surgical procedures are available to correct visual
deficiencies; not all are listed. The procedures described above are
currently the most common. Aircrew members must consult their flight
surgeons before undergoing these procedures.
anatomy and physiology of the eye
Aircrew members are required to understand basic anatomy and
physiology of the eye if they are to use their eyes effectively during
flight. Figure 8-4 shows the basic anatomy of
the human eye.
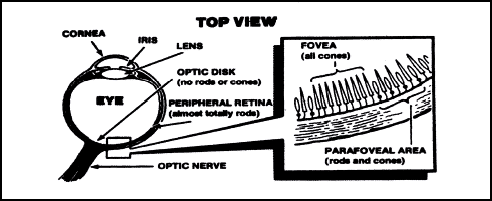
Figure 8-4. Anatomy of the Eye
visual acuity
Visual acuity measures the eye’s ability to resolve spatial
detail. The Snellen visual acuity test is commonly used to measure an
individual’s visual acuity. The Snellen test expresses the comparison
of the distance at which a given set of letters is correctly read to
the distance at which the letters would be read by someone with
clinically normal eyesight. Normal visual acuity is 20/20. A value of
20/80 indicates that an individual reads at 20 feet the letters that
an individual with normal acuity (20/20) reads at 80 feet away. The
human eye functions like a camera. It has an instantaneous field of
view, which is oval and typically measures 120 degrees vertically by
150 degrees horizontally. When two eyes are used for viewing, the
overall FOV measures about 120 degrees vertically by 200 degrees
horizontally.
anatomy and physioloy
When light from an object enters the eye, it passes through
the cornea. The cornea is a circular, transparent protective tissue
that projects forward and protects the eye. Once light travels through
the cornea, it enters the pupil. The pupil is the opening (black centre portion) in the
centre of the iris. The pupil allows the light
to enter the eye to stimulate the retina. The iris is the round,
pigmented (coloured) membrane of the eye surrounding the pupil. For
example, for people with brown, green, or hazel eyes, that coloured
portion is the iris. The iris adjusts the size of the pupil by using
its ciliary muscles, which are attached to the pupil. The iris adjusts
the size of the pupil to regulate the amount of light entering the
eye. When the pupil dilates (enlarges) under low light levels, it
allows more light to enter the eye to further stimulate the retina.
When the pupil constricts (becomes smaller) under high light levels,
it decreases the amount of light entering the eye, avoiding
over saturation (stimulation) of the retina. Light entering the eye is
regulated so that the retina is not under saturated or oversaturated
with light images, which would negatively affect visual acuity. Once
the light travels through the pupil, it will strike the lens. The lens
is a transparent, biconvex membrane located behind the pupil. The lens
then directs (refracts) the light upon the retina (the posterior or
rear portion of the eye). The retina is a complex, structured
membrane, consisting of 10 layers called the Jacob’s membrane. The
retina contains many tiny photoreceptor cells, called rods and cones.
Once light stimulates the retina, it produces a chemical change within
the photoreceptor cells. When the chemical change occurs, nerve
impulses are stimulated and transmitted to the brain via the optic
nerve. The brain deciphers the impulse and creates a mental image that
interprets what the individual is viewing.
retinal photoreceptor
cells
Rods and Cones. The retinal rod and cone cells are so
named because of their shape. The cone cells are used principally for
day or high-intensity light vision (viewing periods or conditions).
The rods are used for night or low-intensity light vision (viewing
periods or conditions). Some of the characteristics of day and night
vision are due to the distribution pattern of rods and cones on the
retina. The centre of the retina, the fovea, contains a very high
concentration of cone cells but no rod cells. The concentration of rod
cells begins to increase toward the periphery of the retina.
Cone Neurology. The retina contains seven million cone
cells. Each cone cell in the fovea is connected to a single nerve
fibre that leads directly to the brain. This single-nerve connection
of each foveal cone to the brain means that each cone generates a
nerve impulse under sufficient light levels. This occurs during
daylight or viewing conditions of high-intensity light exposure. Cone
cells provide sharp visual acuity and the perception of colour. When
crew members view under low light or dark conditions, cone cells
depict shades of black, grey, and white; crew members will perceive
other colours if the light intensity is heightened by artificial light
sources:
Rod Neurology. There are 120 million rod cells in the
retina. Rod cells have a 10-to-1, up to a 10,000-to-1, ratio of rod
cells to neuron cells within the retina. Because of the large number
of rod cells that are connected to each nerve fibre outside of the
fovea, dim light can trigger a nerve impulse to the brain. The
periphery of the retina, where the rods are concentrated, is much more
sensitive to light than is the fovea. This concentration of rods is
responsible for night vision (peripheral vision), which provides for
silhouette recognition of objects. This is also why aircrew members’
eyes are highly sensitive to light when viewing during low ambient
light or dark conditions.
iosopsin and rhodopsin
Vision is possible because of chemical reactions within the
eye. The chemical iodopsin is always present within the cone cells.
Iodopsin permits the cone cells to respond immediately to visual
stimulation, regardless of the level of ambient light. However, rod
cells contain an extremely light-sensitive chemical called rhodopsin,
more commonly referred to as visual purple. Rhodopsin is not always
present in the rods because light bleaches it out and renders the rods
inactive to stimulation. So sensitive is rhodopsin that bright-light
exposure can bleach out all visual purple within seconds.
Night Vision
For night vision to take place, rhodopsin must build up in
the rods. The average time required to gain the greatest sensitivity
is 30 to 45 minutes in a dark environment. When fully sensitized (dark
adapted), the rod cells may become up to 10,000 times more sensitive
than at the start of the dark adaptation period. Through a dilated
pupil, total light sensitivity may increase 100,000 times.
Day Blind Spot
Because humans have two eyes and view all images with
binocular vision, each eye compensates for the day blind spot in the
optic disk of the opposite eye. The day blind spot covers an area of
5.5 to 7.5 degrees. It is located about 15 degrees from the fovea and
originates where the optic nerve attaches to the retina. The size of
the day blind spot is due to the oval shape of the optic nerve
combined with its offset position where it attaches to the retina by
the 5.5 to 7.5 degrees. Where the optic nerve attaches to the retina,
no photoreceptor cells (cones or rods) are present. The day blind spot
only causes difficulty when individuals do not move their head or eyes
but continue to look straightforward while an object is being brought
into the visual field. Figure 8-5 demonstrates
the presence of the day blind spot.
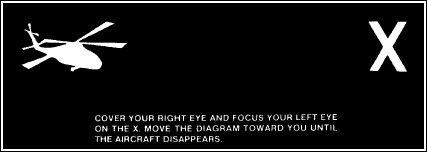
Figure 8-5. Demonstration of the Day Blind Spot
types of vision
The three types of vision (viewing periods) associated with
Army aviation are photopic, mesopic, and scotopic. Each type (viewing
period) requires different sensory stimuli or ambient light
conditions.
photopic vision
Photopic vision, shown in Figure 8-6,
is experienced during daylight or under high levels of artificial
illumination. The cones concentrated in the fovea centralis are
primarily responsible for vision in bright light. Because of the
high-level light condition, rod cells are bleached out and become less
effective. Sharp image interpretation and colour vision are
characteristics of photopic vision. The fovea centralis is
automatically directed toward an object by a visual fixation reflex.
Therefore, under photopic conditions, the eye uses central vision for
interpretation, especially for determining details.
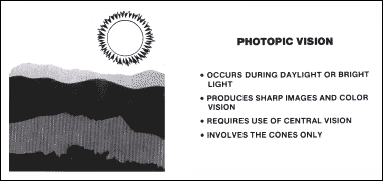
Figure 8-6. Photopic Vision
mesopic vision
8-24. Mesopic vision, shown in Figure 8-7,
is experienced at dawn and dusk and under full moonlight. Vision is
achieved by a combination of rods and cones. Visual acuity steadily
decreases with declining light. Colour vision is reduced (degraded) as
the light level decreases, and the cones become less effective.
Mesopic vision (viewing period) is the most dangerous of all three
types of vision for aircrew members. How degraded the ambient light
condition is during this type of vision will determine what type of
scanning (viewing) technique that aircrew members should use to detect
objects and maintain a safe and incident-free flight. For example,
with the gradual loss of cone sensitivity, off-centre viewing may be
necessary to detect objects in and around the flight path. If aircrew
members fail to recognize the need to change scanning techniques from
central or focal viewing to off-centre viewing, incidents may occur.
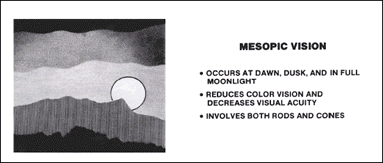
Figure 8-7. Mesopic Vision
scotopic vision
Scotopic vision, shown in Figure 8-8,
is experienced under low-light level environments such as partial
moonlight and starlight conditions. Cones become ineffective, causing
poor resolution of detail. Visual acuity decreases to 20/200 or less,
and color perception is lost. A central blind spot (night blind spot)
occurs when cone-cell sensitivity is lost. Primary colour perception
during scotopic vision is shades of black, grey, and white unless the
light source is high enough in intensity to stimulate the cones.
Peripheral vision is primary for viewing with scotopic vision.
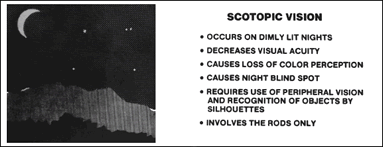
Figure 8-8. Scotopic Vision
Night Blind Spot
The night blind spot, shown in Figure 8-9,
should not be confused with the day blind spot. The night blind spot
occurs when the fovea becomes inactive under low-level light
conditions. The night blind spot involves an area from 5 to 10 degrees
wide in the centre of the visual field. If an object is viewed
directly at night, it may not be seen because of the night blind spot;
if the object is detected, it will fade away when stared at for longer
than two seconds. The size of the night blind spot increases as the
distance between the eyes and the object increases. Therefore, the
night blind spot can hide larger objects as the distance between the
observer and the object increases. Figure 8-10
shows this effect.
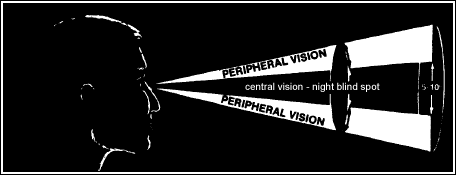
Figure 8-9. Night Blind Spot
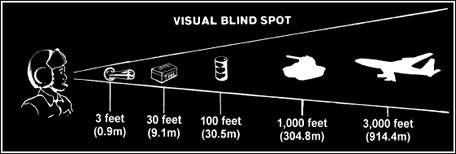
Figure 8-10. Effects of the Night Blind Spot
Peripheral Vision
Stimulation of only rod cells (peripheral vision) is primary
for viewing during scotopic vision. Aircrew members must use
peripheral vision to overcome the effects of scotopic vision.
Peripheral vision enables aircrew members to see dimly lit objects and
maintain visual reference to moving objects. The natural reflex of
looking directly at an object must be reoriented through night-vision
training. To compensate for scotopic vision, aircrew members must use
searching eye movements to locate an object and small eye movements to
retain sight of the object. Aircrew members must use off-centreviewing. Characteristically, if the eyes are held stationary when
focusing on an object for more than two to three seconds using scotopic vision, an image may fade away (bleach out) completely.
factors affecting object
visibility
The ease with which an object can be seen depends on various
factors. Each factor can either increase or decrease the visibility of
an object. The visibility of an object increases as the—
-
Angular size of the object increases as the distance between the
object and the viewer decreases.
-
Illumination (overall brightness) of ambient light increases.
-
Degree of retinal adaptation increases.
-
Colour and contrast between the target and background increase.
-
Position of the target within the visual field (visibility
threshold) increases.
-
The focus of the eye and length of time viewing the object
increase.
-
Atmospheric clarity increases. ND-15 sunglasses can aid
visibility during viewing conditions of excessive light or
brightness.
As aircraft speed increases, there is interference in the
perception of instantaneous visual pictures. In some cases, it may
take one to two seconds or longer to recognize and consciously assess
a complex situation. By the time that an object is eventually
perceived, it may have already been overtaken. The time that it takes
to perceive an object becomes significant to the aircrew member.
Perception time includes the time that it takes—
-
The message indicating that an image of an object has been
identified within the visual field and that image information
travels from the eye to the brain to include the time it takes the
brain to receive, comprehend, and identify the information.
-
The eye to turn toward and focus on the unknown object.
-
An individual to recognize the object and determine its
importance.
-
To transmit a decision to move muscles and cause the aircraft to
respond to control inputs.
dark adaptation
Dark adaptation is the process by which the eyes increase
their sensitivity to low levels of illumination. Rhodopsin (visual
purple) is the substance in the rods responsible for light
sensitivity. The degree of dark adaptation increases as the amount of
visual purple in the rods increases through biochemical reaction. Each
person adapts to darkness in varying degrees and at different rates.
For example, for the person viewing in a darkened movie theatre, the
eye adapts quickly to the prevailing level of illumination. However,
compared to the light level of a moonless night, the light level
within the movie theatre is high. Another example is that a person
requires less time to adapt to complete darkness after viewing in a
darkened theatre than after viewing in a lighted hangar, the lower the
starting level of illumination, the less time is required for
adaptation.
Dark adaptation for optimal night-vision acuity approaches
its maximum level in about 30 to 45 minutes under minimal lighting
conditions. If the eyes are exposed to a bright light after dark
adaptation, their sensitivity is temporarily impaired. The degree of
impairment depends on the intensity and duration of the exposure.
Brief flashes from high-intensity, white (xenon) strobe lights, which
are commonly used as anti-collision lights on aircraft, have little
effect on night vision. This is true because the energy pulses are of
such short duration (milliseconds). Exposure to a flare or a
searchlight longer than one second can seriously impair night vision.
Depending on the brightness (intensity), duration of exposure, or
repeated exposures, an aircrew member’s recovery time to regain
complete dark adaptation could take from several minutes to the full
45 minutes or longer.
Exposure to bright sunlight also has a cumulative and adverse
effect on dark adaptation. Reflective surfaces—such as sand, snow,
water, or man-made structures—intensify this condition. Exposure to
intense sunlight for two to five hours decreases visual sensitivity
for up to five hours. In addition, the rate of dark adaptation and the
degree of night visual acuity decrease. These cumulative effects may
persist for several days.
8-33. The retinal rods are least affected by the wavelength of a
dim red light. Figure 8-11 compares rod and
cone cell sensitivities. Because rods are stimulated by low ambient
light levels, red lights do not significantly impair night vision if
the proper techniques are used. To minimize the adverse effect of red
lights on night vision, crew members should adjust the light intensity
to the lowest usable level and view instruments for only a short time.
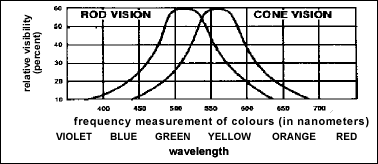
Figure 8-11. Photopic (Cone) and Scotopic (Rod)
Sensitivity to Various Colours
Illness also adversely affects dark adaptation. A fever and
a feeling of unpleasantness are normally associated with illness. High
body temperatures consume oxygen at a higher-than-normal rate. This
oxygen depletion may induce hypoxia and degrade night vision. In
addition, the unpleasant feeling that is associated with sickness is
distracting and may restrict the aircrew member’s ability to
concentrate on flight duties and responsibilities.
night vision protection
Aircrew members should attain maximum dark adaptation in the
minimal possible time. In addition, aircrew members must protect
themselves against the loss of night vision. There are several methods
for accomplishing these requirements.
protective equipment
Sunglasses
When exposed to bright sunlight for prolonged periods,
aircrew members should wear military-issued, neutral-density
sunglasses (ND-15) or equivalent filter lenses when anticipating a
night flight. This precaution minimizes the negative effects of
sunlight (solar glare) on rhodopsin production, which maximizes the
rate of dark adaptation and improves night vision sensitivity and
acuity.
Red-Lens Goggles
Aircrew members, if possible, should wear approved red-lens
goggles or view under red lighting before executing night-flying
operations to achieve complete dark adaptation. This procedure allows
aircrew members to begin dark adaptation in an artificially
illuminated room before flight. Red lighting and red-lens goggles do
not significantly interfere with the production of rhodopsin to
stimulate the effectiveness of the rods for night vision. Red lighting
and red-lens goggles decrease the possibility of undesirable effects
from accidental exposure to bright lights; this is especially true
when aviators are going from the briefing room to the flight line.
Exposure to a bright-light source, however, lengthens the time for
aircrew members wearing red-lens goggles to achieve dark adaptation.
If the light source is high enough in intensity and duration of
exposure is prolonged when viewing with red-lens goggles, aircrew
members will not achieve complete dark adaptation. Red-lens goggles or
red illumination does reduce dark adaptation time and may preserve up
to 90 percent of the dark adaptation in both eyes. Aircrew members
will not use red lighting or red-lens goggles when viewing inside or
outside of the aircraft during flight. Red lighting is a longer
nanometer, which is very fatiguing to the eyes. In addition, for
aircrew members viewing under red lighting, the reds and browns found
on civilian maps not constructed for red-light use will bleach out.
Supplemental Oxygen Equipment
When flying at or above 4,000 feet pressure altitude,
aircrews should use pressure-altitude supplemental oxygen if
available. Adverse effects upon night vision set in at 4,000 feet
pressure altitude. Effective night vision depends on the optimal
function and sensitivity of the retinal rods. Lack of oxygen (hypoxia)
significantly reduces rod sensitivity, increases the time required for
dark adaptation, and decreases night vision.
AR 95-1 describes the requirements of supplemental oxygen use
related to pressure altitudes.
protective measures
Cockpit Light Adjustment
Instrument, cockpit, and rear cargo area overhead lights (if
applicable) should be adjusted to the lowest readable level that
allows instruments, charts, and maps to be interpreted without
prolonged staring or exposure. Although blue-green lighting at low
intensities can also be used in cockpits without significantly
disrupting unaided night vision and dark adaptation, items printed in
blue-green may wash out. The use of blue-green lighting, however, has
several benefits. Blue-green light falls naturally on the retinal wall
and allows the eye to focus easily on maps, approach plates, and
instruments; blue-green lighting results in less eye fatigue. In
addition, the intensity necessary for blue-green lighting is less than
that for red lighting and results in a decreased infrared signature as
well as less glare. When blue-green lighting is used properly, the
decrease in light intensity and the ease of focusing make it more
effective for night vision.
Exterior Light Adjustment
Exterior lights should be dimmed or turned off if possible
and the mission permits. Aviators should consult command policy for
local procedures.
Light-Flash Compensation
Pilots should turn the aircraft away from the light source
if a flash of high-intensity light is expected from a specific
direction. The aircraft should also be manoeuvred away from flares.
When flares are illuminating the viewing area or are inadvertently
ignited nearby, the pilot should manoeuvre to a position along the
periphery of the illuminated area. The aircraft should be turned so
that vision is directed away from the light source. This procedure
minimizes exposure to the light source. When lightning or other
unexpected conditions occur, crew members can preserve their dark
adaptation by covering or closing one eye while using the other eye to
observe. When the light source is no longer present, the eye that was
covered provides the night-vision capability required for flight. If a direct view of the light source cannot
be avoided, cover or close one eye. Remember that dark adaptation
occurs independently in each eye. Depth perception will be severely
degraded or lost, however, because both eyes are no longer completely
dark adapted.
night vision techniques
The human eye functions less efficiently at reduced ambient
light levels. This reduction limits an aircrew member’s visual acuity.
Normal colour vision decreases and finally disappears as the cones
become inactive and the rods begin to function. Tower beacons, runway
lights, or other coloured lights can still be identified if the light
is of sufficient intensity to activate the cones. Normal central
daylight vision also decreases because of the night blind spot that
develops in low illumination or dark viewing conditions. Therefore,
the proper techniques for night-vision viewing must be used to
overcome the reduced visual acuity at lower light levels.
off-centre vision
Viewing an object with central vision during daylight poses
no limitation. If this same technique is used at night, however, the
object may not be seen. This is due to the night blind spot that
exists under low light illumination. To compensate for this
limitation, off-centre vision must be used. Figure
8-12 illustrates the off-centre vision technique. With this
technique, crew members view an object by looking 10 degrees above,
below, or to either side rather than directly at the object. Thus, the
eyes can maintain visual contact with an object via peripheral vision.
Aircrew members should avoid viewing objects for either too short or
too long a time.
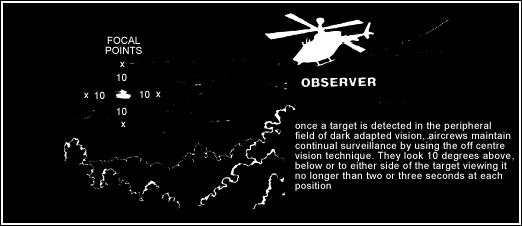
Figure 8-12. Off-Centre Vision Technique
Rapid head or eye movements and fixations decrease the
integrating capability of the dark-adapted eye. A steady fixation
lasting one-half to one second achieves the maximum sensitivity.
An object viewed longer than two to three seconds tends to
bleach out and become one solid tone. Therefore, the object can no
longer be seen. This creates a potentially unsafe operating condition.
The aircrew member must be aware of the phenomenon and avoid viewing
an object longer than two to three seconds. By shifting the eyes from
one off-centre point to another, the aircrew member can see the object
in the peripheral field of vision.
scanning
During daylight, objects can be perceived at a great
distance with good detail. At night, the range is limited and detail
is poor. Objects along the flight path can be more readily identified
at night when aircrew members use the proper techniques to scan the
terrain. To scan effectively, aircrew members look from right to left
or left to right. They should begin scanning at the greatest distance
at which an object can be perceived (top) and move inward toward the
position of the aircraft (bottom). Figure 8-13
shows this scanning pattern. Because the light-sensitive elements of
the retina are unable to perceive images that are in motion, a
stop-turn-stop-turn motion should be used. For each stop, an area
about 30 degrees wide should be scanned. This viewing angle will
include an area about 250 meters wide at a distance of 500 meters. The
duration of each stop is based on the degree of detail that is
required, but no stop should last more than two or three seconds. When
moving from one viewing point to the next, aircrew members should
overlap the previous field of view by 10 degrees. This scanning
technique allows greater clarity in observing the periphery. Other
scanning techniques, as illustrated in Figure 8-14,
may be developed to fit the situation.
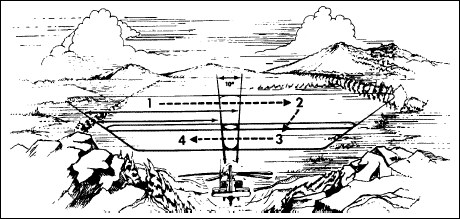
Figure 8-13. Scanning Pattern
shapes or silhouettes
Because visual acuity is reduced at night, objects must be
identified by their shapes or silhouettes. To use this technique, the
aircrew member must be familiar with the architectural design of
structures in the area covered by the mission. A silhouette of a
building with a high roof and steeple can easily by recognized as a
church in the United States. However, religious buildings in other
parts of the world may have low-pitched roofs with no distinguishing
features, to include cylinder-shaped structures. For example, the
cylinder-shaped structures attached to Muslim mosques (religious
temples), called minarets, are similar in shape to the silos attached
to barns in the United States. Features depicted on the map will also
aid in recognizing silhouettes.
distance estimation and
depth perception
The cues to distance estimation and depth perception are
easy to recognize when aircrew members use central vision under good
illumination. As light levels decrease, their ability to judge
distance accurately degrades and their eyes are vulnerable to
illusions. Aircrew members can better judge distance at night if they
understand the mechanisms of visual cues related to distance
estimation and depth perception. Distance can be estimated by using
individual cues or by using a variety of cues. Aircrew members
normally use subconscious factors to determine distance. They can more
accurately estimate distance if they understand those factors and then
learn to look for or be aware of other distance cues. These cues to
distance or depth perception may be monocular or binocular.
binocular cues
Binocular cues depend on the slightly different view each
eye has of an object. Thus, binocular perception is of value only when
the object is close enough to make a perceptible difference in the
viewing angle of both eyes. In the flight environment, most distances
outside the cockpit are so great that the binocular cues are of
little, if any, value. In addition, binocular cues operate on a more
subconscious level than do monocular cues. Study and training will not
greatly improve them; therefore, they are not covered in this
publication.
Monocular
cues
Several monocular cues aid in distance estimation and depth
perception. These cues are geometric perspective, motion parallax,
retinal image size, and aerial perspective. They can be remembered by
the acronym GRAM.
Geometric Perspective
An object appears to have a different shape when crew
members view it at varying distances and from different angles. The
types of geometric perspective include linear perspective, apparent
foreshortening, and vertical position in the field.
Figure 8-15 illustrates these. They can be remembered by the
acronym LAV.
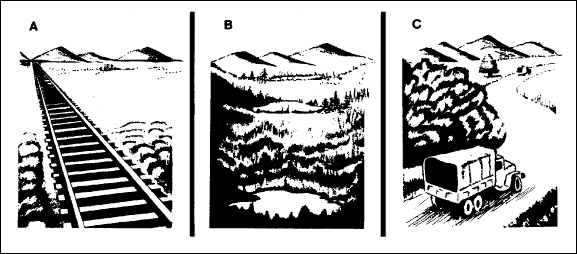
Figure 8-15. Geometric Perspective
Linear Perspective. Parallel lines, such as railroad
tracks, tend to converge as distance from the observer increases. This
is illustrated in part A of Figure 8-15.
Apparent Foreshortening. The true shape of an object
or terrain feature appears elliptical (oval and narrowed appearance)
when viewed from a distance when aircrew members are flying at both
higher and lower altitudes. As the distance to the object or terrain
feature decreases, the apparent perspective changes to its true shape
or form. When flying at lower altitudes and viewing at greater
distances, aircrew members may not view objects clearly. If the
mission permits, pilots should gain altitude and decrease distance
from the viewing area to compensate for this perspective. That is,
once the aircraft increases in altitude and distance between the
aircraft and the viewing area decreases, the viewing field widens and
enlarges so that objects within that field of view become apparent.
Part B of Figure 8-15 illustrates how the shape
of a body of water changes when viewed at different distances while
the aircraft maintains the same altitude.
Vertical Position in the Field. Objects or terrain
features that are at greater distances from the observer appear higher
on the horizon than do those that are closer to the observer. In part
C of Figure 8-15, the higher vehicle appears to
be closer to the top and is judged as being at a greater distance from
the observer. Before flight, aircrew members should already be
familiar with the actual sizes, heights, or altitudes of known objects
or terrain features within and around the planned flight route. If the
situation and time permit, aircrew members can reference published
information to verify actual sizes, heights of objects, and terrain
features within their flight path. In addition, the aircrew members
should cross-reference their aircraft’s altitude indicator to confirm
that actual aircraft altitude is adequate to safely negotiate the
object or terrain feature without prematurely changing the aircraft’s
heading, altitude, or attitude or a combination of these.
Motion Parallax
This is often considered the most important cue to depth
perception. Motion parallax refers to the apparent, relative motion of
stationary objects as viewed by an observer who is moving across the
landscape. Near objects appear to move past or opposite the path of
motion; far objects seem to move in the direction of motion or remain
fixed. The rate of apparent movement depends on the distance that the
observer is from the objects. Objects near the aircraft appear to move
rapidly, while distant objects appear to be almost stationary. Thus,
objects that appear to be moving rapidly are judged to be near while
those moving slowly are judged to be at a greater distance. Motion
parallax can be apparent during flight. One example is an aircraft
flying at 5,000 feet AGL. At that altitude, the terrain off in the
distance appears to be stationary. The terrain immediately below and
to either side of the aircraft may appear to be moving slowly,
depending on the forward airspeed of the aircraft. The opposite is
true when an aircraft descends to 80 feet AHO with a forward airspeed
of 120 knots. The terrain and objects in the horizon appear to move at
a faster rate, while the terrain and objects underneath and to either
side of the aircraft appear to pass by at a high rate of speed.
Retinal Image Size
Distance Estimation. An image focused on the retina
is perceived by the brain to be of a given size. The factors that aid
in determining distance using the retinal image are known size of
objects, increasing and decreasing size of objects, terrestrial
association, and overlapping contours or interposition of objects.
These factors can be remembered by the acronym KITO.
Known Size of Objects. The nearer an object is to the
observer, the larger its retinal image. By experience, the brain
learns to estimate the distance of familiar objects by the size of
their retinal image. Figure 8-16 shows how this
method is used. A structure projects a specific angle on the retina,
based on its distance from the observer. If the angle is small, the
observer judges the structure to be at a great distance. A larger
angle indicates to the observer that the structure is close. To use
this cue, the observer must know the actual size of the object and
have prior visual experience with it. If no experience exists, aircrew
members determine the distance to an object primarily by motion
parallax.
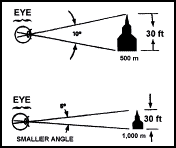
Figure 8-16. Known Size of Object Used to Determine
Distance
Increasing or Decreasing Size of Objects. If the
retinal image of an object increases in size, the object is moving
closer to the observer. If the retinal image decreases, the object is
moving farther away. If the retinal image is constant, the object is
at a fixed distance.
Terrestrial Association. Comparison of one object,
such as an airfield, with another object of known size, such as a
helicopter, will help to determine the relative size and apparent
distance of the object from the observer. Figure
8-17 shows that that objects ordinarily associated together are
judged to be at about the same distance. For example, a helicopter
that is observed near an airport is judged to be in the traffic
pattern and, therefore, at about the same distance as the airfield.
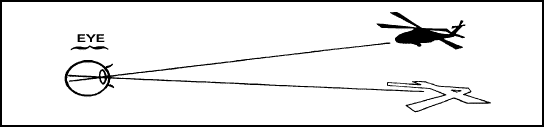
Figure 8-17. Terrestrial Association of Objects Used
to Determine Distance
8-60. Overlapping Contours or Interposition of Objects.
When objects overlap, the overlapped object is farther away. For
example, an object partly concealed by another object is behind the
object that is concealing it. Aircrew members must be especially
conscious of this cue when making an approach for landing at night.
Lights disappearing or flickering in the landing area should be
treated as barriers, and the flight path should be adjusted
accordingly. Figure 8-18 illustrates
overlapping contour.
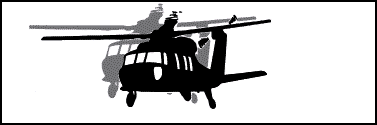
Figure 8-18. Overlapping Contour Used to Determine
Distance
Aerial Perspective
The clarity of an object and the shadow cast by it are
perceived by the brain and are cues for estimating distance. To
determine distance with these aerial perspectives, aircrew members use
the factors discussed below.
Fading of Colours or Shades. Objects viewed through
haze, fog, or smoke are seen less distinctly and appear to be at a
greater distance than they actually are. If atmospheric transmission
of light is unrestricted, an object is seen more distinctly and
appears to be closer than it actually is. For example, the cargo
helicopter in Figure 8-19 is larger than the
observation helicopter, but because of the difference in viewing
distance and size, they both project the same angle on the observer’s
retina. From this cue alone, assuming the observer has no previous
experience with their appearance, both helicopters appear to be the
same size. However, if the cargo helicopter is known to be a larger
aircraft but is seen less distinctly because of visibility
restrictions, it would be judged to be farther away and larger than
the observation helicopter. Another example is that aircrew members
may not be able to distinguish green from red anti-collision lights and
the actual interval between aircraft when an additional aircraft is
operating at a distance. Both lights may appear to be white, and in
addition, they may even blend in with the surrounding foreground.
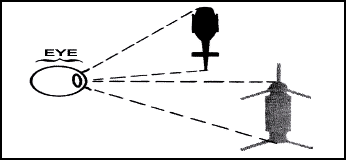
Figure 8-19. Fading Colour or Shade Used to Determine
Distance
Loss of Detail or Texture. The farther from an object
that an observer is, the less apparent discrete details become. For
example, a cornfield at a distance becomes a solid colour, leaves and
branches of a tree become a solid mass, and objects are judged to be
at a great distance. With the aircraft operating on the ground, crew
members view the grass or gravel immediately below, in front of, or
alongside the aircraft. As the aircraft slowly ascends, they maintain
a view of that grass or gravel. Aircrew members will notice that, as
the aircraft ascends, the clarity and detail of the grass is fading
and eventually blends in with the terrain as a whole, causing the
viewer not to be able to identify blades of grass or gravel.
Environmental factors increase the effects of degraded texture and
detail of objects throughout the visual field. This loss of detail, in
turn, severely decreases depth perception and is a contributing factor
in relation to aircrew members’ misjudgements of what is seen or not
seen and the occurrence of incidents related to those misjudgements.
Position of Light Source and Direction of Shadow.
Every object will cast a shadow if there is a source of light. The
direction in which the shadow is cast depends on the position of the
light source. If the shadow is cast toward the observer, the object is
closer than the light source to the observer.
Figure 8-20 shows how light and shadow help determine distance.
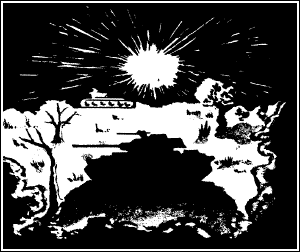
Figure 8-20. Position of Light Source and Direction
of Shadow Used to Determine Distance
visual illusion
As visual information decreases, the probability of spatial
disorientation increases. Reduced visual references also create
several illusions that can cause spatial disorientation.
metrological condition
and night vision
Although a flight may begin with clear skies and
unrestricted visibility, meteorological conditions may deteriorate
during flight. Because of reduced vision at night, clouds can appear
gradually and easily go undetected by aircrew members. The aircraft
may even enter the clouds inadvertently and without warning. At low
altitudes, fog and haze can be encountered. Visibility can deteriorate
gradually or suddenly. Because it is difficult to detect adverse
weather at night, crew members should be constantly aware of changing
weather conditions. The following conditions are indicators of adverse
weather at night.
8-67. The ambient light level is gradually reduced as cloud
coverage increases. Visual acuity and contrast of terrain features are
lost, possibly to complete obscurity. If this condition should occur,
pilots should initiate inadvertent IMC procedures. Aircrew members
must follow their local SOPs and command directives and realize that
inadvertent IMC at night is one of the leading causes of Class A
mishaps.
8-68. If the moon and stars cannot be seen, clouds are present. The
less visible the stars and moon, the heavier the cloud coverage.
8-69. Clouds obscuring the illumination of the moon create shadows.
These shadows can be detected by observing the varying levels of
ambient light along the flight route.
8-70. The halo effect, which is observed around ground lights,
indicates the presence of moisture and possible ground fog. As the fog
and moisture increase, the intensity of the lights will decrease. This
same effect is apparent during flight. As moisture increases, the
light that is emitted from the aircraft is reflected back upon the
aircraft. When this reflection occurs, it is possible to misjudge
terrain features, man-made structures, and the actual position,
heading, and altitude of other aircraft including the layout and
height of the terrain below.
8-71. The presence of fog over water surfaces indicates that the
temperature and dew point are equal. It also indicates that fog may
soon form over ground areas.
self imposed stress and vision
The normal aviation stress that aircrew members experience
in flight, such as altitude, may not be controllable and may affect
mission performance somewhat. In addition, those involved in aviation
must cope with self-imposed stress. Unlike aviation stress, aircrew
members themselves can control self-imposed stress. The factors that
cause this stress are drugs, exhaustion, alcohol, tobacco, and hypoglycemia and nutrition. These factors, shown in
Figure 8-21, can be remembered by the acronym DEATH.
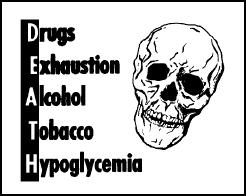
Figure 8-21. Self-Imposed Stress Factors
drugs
Adverse side effects associated with drug use are illness
and degradation in motor skills, awareness level, and reaction time.
Aircrew members who become ill should consult the flight surgeon. Crew
members should avoid self-medicating; it is unauthorized for flight
personnel.
exhaustion
Tiredness reduces mental alertness. In situations that
require immediate reaction, exhaustion causes aircrew members to
respond more slowly. They tend to concentrate on one aspect of a
situation without considering the total environment. Rather than use
proper scanning techniques, they are prone to stare, which may cause
incidents. Good physical conditioning should decrease fatigue and
improve night-scanning efficiency. However, excessive exercise in a
given day can lead to increased fatigue. Night flying is more
stressful than day flying. Aircrew members should follow prescribed
crew-rest policies. Multiple factors cause exhaustion; normally,
exhaustion does not set in from one factor alone. Contributing factors
associated with exhaustion include poor diet habits, lack of rest,
poor sleeping patterns, poor physical condition, an inadequate
exercise routine, environmental factors, dehydration, and combat
stress. In combination, these can create exhaustion. Common side
effects associated with exhaustion include altered levels of
concentration, awareness, and attentiveness; increased drowsiness
(nodding off or falling asleep); and ineffective night-vision viewing
techniques (staring, rather than scanning).
alcohol
Alcohol causes a person to become uncoordinated and impairs
judgment. It hinders the aircrew member’s ability to view properly.
The aircrew member is likely to stare at objects and neglect proper
scanning techniques, particularly at night. In addition, as is
indicated by the physiological response of the body to a hangover, the
effects of alcohol are long lasting. Alcohol induces histotoxic
hypoxia, which is the poisoning of the bloodstream, interfering with
the use of oxygen by body tissues. One ounce of alcohol in the
bloodstream at sea level places an individual at 2,000 feet
physiologically. Every ounce of alcohol in the bloodstream at sea
level increases the body’s physiological altitude. For example, an
individual who consumes three ounces of alcohol at sea level and is
then placed at 4,000 feet actual pressure altitude has a physiological
altitude of 10,000 feet. Now, combined with the histotoxic hypoxia
effects is hypoxic hypoxia. This individual’s time of useful
consciousness is severely impaired. If the flight is longer than 60
minutes, the individual may become unconscious and may even die from
lack of oxygen. The guidance for performing or resuming aircrew member duties
when alcohol is involved is 12 hours after the last consumed alcohol
with no residual physiological effects present. Aircrew member duties
include preflight and postflight actions, to include maintenance; they
are not limited to actual operation of the aircraft or flight.
Detrimental effects associated with the consumption of alcohol include
poor judgment, decision making, perception, reaction time,
coordination, and scanning techniques (tendency to stare at objects).
tobacco
Of all self-imposed stresses, cigarette smoking decreases
visual sensitivity at night the most. The haemoglobin of the red blood
cells has a 200 to 300 times greater affinity for carbon monoxide than
for oxygen. That is, the haemoglobin accepts the carbon monoxide far
more rapidly than it will accept oxygen. During normal pulmonary
perfusion (gas exchange within the lungs), carbon dioxide is released
from the bloodstream when an individual exhales. When an individual
inhales, the normal action is that oxygen is absorbed into the blood (haemoglobin
of the red blood cell); thus, normal levels of oxygen and other gas
levels are being maintained within the bloodstream. Smoking increases
CO, which in turn, reduces the capacity of the blood to carry oxygen.
The hypoxia that results from this increase in carbon monoxide is
hypaemic hypoxia, which negatively affects the aircrew member’s
peripheral vision and dark adaptation. If, for example, an individual
smokes 3 cigarettes in rapid succession or 20 to 40 cigarettes within
a 24-hour period, the carbon monoxide content of the blood is raised 8
to 10 percent. The physiological effect at ground level is the same as
flying at 5,000 feet. More importantly, the smoker has lost about 20
percent of night-vision capability at sea level.
Table 8-1 compares reduced night vision at varying altitudes for
smokers and nonsmokers.
Table 8-1. Percentage Reduction of Night Vision at
Varying Altitudes for Smokers and Nonsmokers
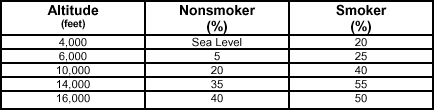
hypoglyemia and nutritional deficiency
Aviation personnel should avoid missing or postponing meals.
They should also avoid supplementing primary meals with fast sugars
(for example, sodas and candy bars). These foods and beverages can
cause low blood-sugar levels. Low blood-sugar levels may result in
hunger pangs, distraction, a breakdown in habit patterns, a shortened
attention span, and other physiological changes. Supplementing with
fast sugars as the primary diet will, on average, sustain the
individual for up to 30 to 45 minutes. The negative effects will then
increase in intensity. Not only can an improper diet cause
hypoglycaemia, but a diet that is deficient in Vitamin A can also
impair night vision. Vitamin A is an essential element in the build-up
of rhodopsin (visual purple) for stimulation of the rod cells. Without
this build-up of rhodopsin, night vision is severely degraded. An
adequate intake of Vitamin A—through a balanced diet that includes
such foods as eggs, butter, cheese, liver, carrots, and most green
vegetables—will help maintain visual acuity. Aircrew members must
consult a flight surgeon before consuming Vitamin A supplements that
are not organic to the foods noted above.
flight hazards
Solar glare and bird strikes are possible hazards that an
aircrew member may encounter during low-level flight.
solar glare
Glare from direct, reflected, or scattered sunlight causes
discomfort and reduces visual acuity. To reduce or eliminate
discomfort, every aircrew member should wear, lowered, the tinted
visor or wear issued ND-15 sunglasses with the clear visor. Day
blindness can occur in areas of extreme solar glare (in snow, over
water, or in desert environments).
bird strikes
This hazard can occur during the day or at night during
low-level flight. Cockpit windshields are designed to withstand
impacts, but the potential exists for shattering. According to the FAA,
if an aircraft travelling at an airspeed equivalent to a
120-mile-per-hour ground speed strikes a two-pound seagull, the force
exerted would be equal to 4,800 pounds. Therefore, the clear visor for night flights and
the tinted visor for day flights (if the viewing environment warrants)
should be worn (lowered) by aircrew members. These visors would not
only protect their eyes from the remains of the bird but also, more
importantly, from the glass fragments of the windshield.
principles of proper
vision
Aircrew members must completely understand the function of
the eye and the techniques that they can employ to overcome visual
limitations. It is usually not the lack of visual acuity that causes
problems for aircrew members but rather a lack of understanding of
"how to see" properly. In summary, the principles of proper vision
require that aircrew members—
-
Understand the types of vision and the limitations of each and
that visual acuity will normally be lost under low levels of
illumination.
-
If corrective lens are prescribed
to aircrew members, they must use corrective lens (glasses) in all
modes of flight.
-
Be aware that it will take 30 to 45 minutes for the average
individual’s eyes to reach maximum dark adaptation.
-
Remember to use off-centre vision when viewing objects under
reduced lighting conditions.
-
Use supplemental oxygen, if available, on flights (especially
night flights) at or above 4,000 feet pressure altitude.
-
Avoid self-imposed stress.
-
Protect night vision by avoiding bright lights once dark
adaptation has been achieved.
-
Scan constantly when viewing objects outside the cockpit, day or
night.
|